Abstract
In recent years, halide perovskite nanostructures have had great advances and have opened up a bright future for micro/nanolasers. However, upconversion lasing by two-photon excitation with mode selection and high quality factor in one device is still rarely reported. Herein, two lasing modes are demonstrated in the all-inorganic perovskite microplates with subwavelength thickness and uniform square shape. The net optical gain is quickly established in less than 1 ps and persists more than 30 ps, revealed by ultrafast transient absorption spectroscopy. The temperature-dependent low-threshold amplified spontaneous emission confirms the net gain for stimulated emission with a high characteristic temperature of 403 K, far surpassing the all-inorganic semiconductor gain media. Remarkably, upconversion lasing based on two kinds of microcavity effects, Fabry–Pérot and whispering-gallery modes, from the microplates at room temperature is successfully achieved with a low threshold operating in multi- or single-mode, respectively. Surprisingly, the quality factor () is among the best values obtained from perovskite micro/nanoplate upconversion lasers without an external cavity. Moreover, the highly stable chromaticity with color drift only less than 0.1 nm also outbalances the all-inorganic ones. These superior performances of microplate lasing with a facile solution synthesis procedure will offer a feasible structure to fabricate specific functionalities for high-performance frequency upconversion micro/nanoscale photonic integrated devices.
1. INTRODUCTION
The miniaturization and integration of photonic components in nano/micrometer-scale proximity on chip have boosted integrated information processing systems [1]. As an important component, great advances have been demonstrated in miniaturized optical sources for optoelectronic integration, sensing, and optical communications [1–3]. In the past few years, metal trihalide perovskites () have been demonstrated with excellent performance in miniaturized lasing devices due to their unique photoelectric properties, such as high optical gain, large absorption coefficient, and narrow linewidth [4–8]. For instance, perovskite lasing has been demonstrated with various microcavity structures, such as Fabry–Pérot (F-P) lasing in micro/nanowires and micro/nanocubes, and whispering-gallery-mode (WGM) lasing in micro/nanoplates and micro/nanospheres [9–16]. However, both of these two lasing modes, i.e., F-P and WGM, realized in the same structure, have been rarely studied. Polygon microcavities, such as micro/nanoplates, have multiple corners and boundaries [14], indicating their emission patterns of multiple directional emissions, promising for mode selection lasing actions.
Compared with single-photon excitation, two-photon pumped lasers without phase-matching requirements have many attractive merits, such as large penetration depth, high spatial resolution, and reduced photodamage, rendering in optical storage, infrared detection, and medical imaging [17–19]. Recently, much progress has been made in two-photon pumped lasing in perovskite nanostructures. For nanocrystals, the two-photon pumped F-P, WGM, and random lasing have been demonstrated in microtubules, spheres, or distributed Bragg reflectors with threshold of and quality factor of [20–23]. Different from the necessary external cavity in nanocrystal lasing with large mode volume, the single nanostructure could support lasing oscillation in nanowires and nanoplates. For nanowires and nanoplates, the two-photon pumped F-P mode oscillation between two facets and WGM oscillation among four facets have also been realized, respectively [24–31]. However, most of the two-photon pumped lasing devices operate in multimode with linewidth , which severely lowered the coherence and monochromaticity of the coherent optical sources. Therefore, directly obtaining high-quality single- or multimode lasing in one device has great promise for optoelectronic integration, which could decrease the demand of the complexity in cavity configuration.
More recently, a novel member of the perovskite family, all-inorganic , has a unique two-dimensional (2D) electrical structure composed of a cation between layers, which has been recognized as promising for applications in solar cells, photodetectors, and light-emitting devices [32–35]. The reduced dimension of 2D structure will have high optical gain, enhanced absorption cross section, and increased exciton binding energy [36,37], bringing huge benefits for lasing.
Sign up for Photonics Research TOC. Get the latest issue of Photonics Research delivered right to you!Sign up now
Herein, we explicate the mechanism of optical gain from perovskite microplates, which establishes rapidly in less than 1 ps and lasts more than 30 ps. Subsequently, temperature-dependent amplified spontaneous emission (ASE) is observed with low threshold and high characteristic temperature of 403 K. Remarkably, the frequency-upconverted lasing with low thresholds and high quality factors operating in F-P mode or WGM is demonstrated from a pristine microplate at room temperature. Most importantly, the obtained quality factor () is the highest value obtained in upconversion perovskite micro/nanoplate lasings to our knowledge. All these findings suggest that perovskite microplates will provide versatile opportunities and stable platforms for the exploration of nonlinear nanophotonic integrated devices.
2. EXPERIMENT
A. Preparation and Synthesis of Microplates
Lead (II) bromide (, 99%, Xi’an Polymer Light Technology Corp.), cesium carbonate (, 99.9%, Adamas), oleic acid (OA, 90%, Sigma Aldrich), oleylamine (OLAM, 70%, Sigma Aldrich), 1-octadecene (ODE, 90%, Sigma Aldrich) were used. All chemicals were used without further purification in preparation of microplates. Cesium stock solution was obtained by mixing 100 mg , 0.4 mL OA, and 3.75 mL ODE in a 100 mL three-necked flask and degassing under nitrogen () flow at 120°C for 1 h until mixed in a 100 mL three-necked flask placed in a heating jacket with , and then heated to 120°C under . Temperature was set up to 135°C. 0.3 mL OLAM and 0.4 mL OA were injected into the crude solution quickly and the temperature of 135°C was maintained for 1 h until the was solubilized completely. 0.4 mL Cs-OA solution was swiftly injected and stirred for 2 h, and then was cooled by the ice bath. Finally, microplates were obtained after several purification processes and dispersed in toluene for testing.
B. Characterization of Microplates
The scanning emission microscopy (SEM) image was recorded using a ZEISS LIBRA 200FE microscope. The crystal phase of the sample was characterized by X-ray diffraction (XRD) with CuKa radiation (XRD-6100, SHIMADZU, Japan). The linear absorption spectrum was obtained by a scan UV–Vis spectrophotometer (UV–Vis: UV-2100, Shimadzu, Japan).
C. Ultrafast Transient Absorption Measurements
A femtosecond amplified Ti:sapphire laser source was used as the laser source with pulse duration of , repetition rate of 1 kHz, and central wavelength of 800 nm. The laser output is divided into two parts, and the major part is used to generate pump pulses at 355 nm () in an optical parametric amplifier. The pump beam is modulated at 500 Hz by a mechanical chopper. Another part of the laser is used to generate white light through a crystal plate. The white light can be focused onto the sample by an off-axis parabolic mirror and detected by a silicon detector. The time delay between pump and probe beams is controlled by a motorized stage. All experimental data are corrected for the chirp induced by the nonlinear process in white-light generation.
D. Temperature-Dependent ASE Experiments
For the two-photon-pumped ASE experiments, a commercial Ti:sapphire laser system with repetition rate of 1 kHz and pulse width of 35 fs was employed as the excitation source. The sample was put in a cryogenic sample chamber (MicrostatN2, Oxford Instruments), and the laser beam was focused by a lens (focus length: 100 mm). The photoluminescence (PL) spectra are measured at temperatures from 100 K to 300 K in a vacuum. A variable neutral density filter was employed to adjust the pump intensity on the sample, and the varied power is measured with a power meter. The adjustable slit is used to adjust the width of the excitation beam. Then the pump-intensity-dependent PL signal is collected from the sample by a backscattering geometry and the residual excitation light is blocked by a band-pass filter.
E. Lasing Characterization
The laser beam was focused by a microscope objective (MPLFLN , ) to excite the single microplate, and the emission signal was collected from the top of a micro-PL system by the same objective and detected by a spectrograph (Acton SpectraPro SP-2358, Princeton Instruments) and a silicon charge coupled device with a resolution of 0.04 nm.
3. RESULTS AND DISCUSSION
The perovskite microplates are synthesized by the improved solution process [32]. As investigated by the SEM image shown in Fig. 1(a), the perovskite displays the morphology of a smooth and regular square in an average length of about 4 μm and thickness of about 400 nm. Interestingly, the 2D structure of demonstrates a unique layer stack structure in which the layer and layer are alternately stacked as displayed in Fig. 1(b) [32,38]. Moreover, Fig. 1(c) presents the crystalline phase and purity of the obtained microplates by X-ray diffraction (XRD). The major diffraction peaks at , 20.9, 23.4, 29.3, 35.4, 37.9, and 47.6 (47.9) deg that correspond to (002), (200), (210), (213), (312), (313), and (413)/(420) planes can be well assigned to the standard powder XRD patterns of pure tetragonal (PDF#25-0211) with no additional diffraction peaks of . The emission spectra under excited wavelengths at 800 nm centered at with full width at half-maximum (FWHM) of and optical absorption spectrum are depicted in Fig. 1(d). A broad absorption band below band gap is observed, which may be ascribed to the localized states arising from the structural disorder or low crystallinity [39]. To further confirm the two-photon process, the intensity dependence of PL spectra under two-photon excitation is investigated. The log–log plots of the pump-fluence-dependent integrated PL intensity (Fig. 6 in Appendix A.1) can be fitted linearly with a slope of 1.91, clearly indicating that the two-photon absorption actually occurs.
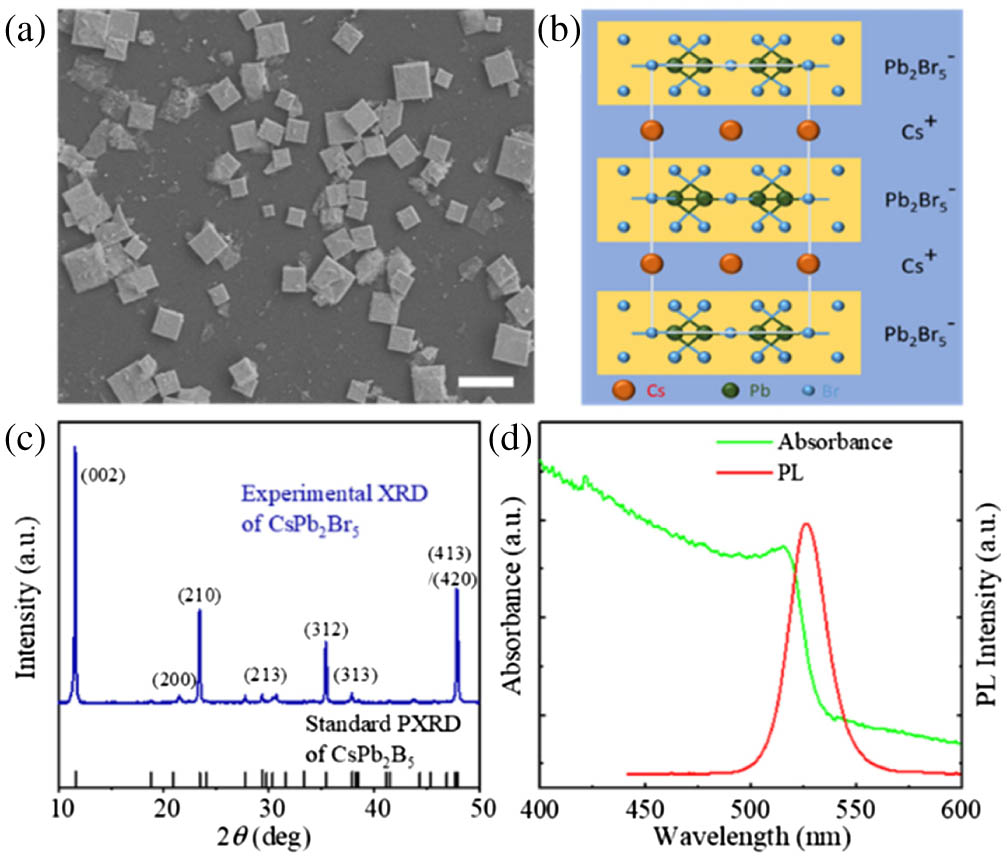
Figure 1.Optical characterizations of perovskite microplates. (a) SEM image. The scale bar is 10 μm. (b) Schematic crystal structure. (c) Experimental and standard powder XRD patterns of tetragonal . (d) Linear absorption (green) and PL spectrum (red).
Population inversion is a fundamental prerequisite for lasing. When electrons are pumped into high excited states by absorbing photons, the optical gain could be possible if the population inversion is established when the accumulation of the emissive states is faster than the escaping processes. To track the gain dynamics, the ultrafast transient absorption (TA) spectroscopy is implemented. Figures 2(a) and 2(b) present the pseudo-color and corresponding TA spectra at different delay times of perovskite under strong fluence excitation, showing four distinct spectral features. From high energy to low energy, a long-lived photo-induced absorption () at the short wavelength () contributed from the absorption of the excited states, and a strong photobleaching (PB) band arising from the band filling effect at 500–530 nm could be observed. Notably, a photo-induced absorption () at the low energy at early times was observed, subsequently rapidly evolving to a negative signature around 540 nm, which could be assigned to the stimulated emission (SE) [20,40]. In addition, we observed an obvious redshift of the PB peak position indicated by the red arrow in Fig. 2(b), which could be ascribed to the trade-off between the redshift produced by the band-gap renormalization and the blueshift induced by the Burstein–Moss effect [41].
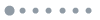
Figure 2.TA spectra of perovskite . (a) 2D pseudo-color TA spectra. (b) TA spectra at different delay times. (c) The kinetics of prominent PB signal. (d) The kinetics of SE. Inset: a magnified view of the spectroscopic signature of SE before 11 ps.
Due to the excess energy, hot carrier cooling will play an essential role in determining the performance of optoelectronic devices. As shown in Fig. 2(c), the formation of a PB signal within the first 2 ps with a rising constant of 480 fs is observed, indicating the fast decay of hot carriers from the high-energy level to the band edge. This rapid process would boost effectively the buildup of population inversion for SE. To further analyze the temporal dynamics of optical gain, the TA signal probed at 540 nm is plotted in Fig. 2(d). At early time in the inset, a strong PA is first observed with decay lifetime of 559 fs and then replaced by a strong negative feature, indicating the onset of SE. Consequently, the lifetime of optical gain of is extracted. With further increasing the pump fluence, the SE in Fig. 7 in Appendix A.2 displays a slightly slow buildup due to the high carrier density. Simultaneously, the optical gain persists longer and gain saturation occurs. The fast buildup and persistence for more than 30 ps of net gain from the microplates would hold great promise for light amplification.
To further demonstrate the gain properties, we explore the temperature-dependent ASE under two-photon excitation of perovskite microplates. As shown in Fig. 3(a), the emission spectra at 300 K are recorded under different excitation densities, which display a significant gain-induced increase in emission intensity and linewidth narrowing. The detail evolution of FWHM and threshold behavior in Fig. 3(b) provide the clear evidence of ASE. In other words, as the pump fluence increases, the PL intensity increases nonlinearly and FWHM decreases quickly from to with a distinct knee at (ASE threshold). Notably, the ASE peak that emerges at well corresponds to the gain revealed by ultrafast TA spectroscopy. Figure 3(c) presents the emission intensity with respect to the ASE action of microplates from 100 K to 300 K under a two-photon pump confirmed by the super-linear increase behaviors. The ASE thresholds display a slight increase with temperature, depicted in Fig. 3(d), which is commonly observed in semiconductors [42]. To further describe the ASE properties, the threshold action can be analyzed by the following equation [42]: in which is the characteristic temperature to indicate the capacity of the temperature-dependent ASE threshold. From Eq. (1), a high of 403 K is extracted, suggesting that the ASE threshold is highly insensitive to temperature variation, far surpassing the all inorganic and bulk-based semiconductor lasers [12,42,43]. Moreover, the long-term ASE operation at room temperature is recorded in Fig. 8 in Appendix A.3. After uninterrupted excitation for longer than 150 min or over excitation cycles, the ASE intensity still shows a stable trend. Thus, a microplate could be used for temperature-insensitive and stable two-photon-excited upconversion devices.
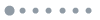
Figure 3.Temperature-dependent ASE actions from microplates. (a) The PL spectra of two-photon pumped ASE at 300 K. Inset: photograph of the microplate excited above ASE threshold by a cylindrical lens. (b) The integrated intensity and FWHM with respect to pump intensity. (c) Normalized PL intensity as a function of excitation intensity at different temperatures. (d) Pump threshold intensity versus temperature. The solid red line is the fit according to Eq. (1).
Two-photon pumped lasers have been considered a promising strategy to achieve frequency upconversion, and they show promising nonlinear optical performances. In the following, we study the frequency upconversion application for lasers from microplates at room temperature. For a pristine perovskite nanostructure, F-P lasers and WGM lasers have been demonstrated in micro/nanowires and micro/nanoplates by virtue of their superior optical gain, respectively [9,14]. Owing to the smooth and regular morphology of microplates and the difference of refractive index between perovskite and its surroundings, the cavity modes inside could resonate following the unidirectional F-P mode between two facets and WGM among the four corners by total internal reflection.
First, the excitation laser beam is focused on the edge of a single microplate. The total spectra with increasing pump fluence are shown in Fig. 9 in Appendix A.4. Figure 4(a) presents the selected pump-intensity-dependent emission spectra from a single microplate, which clearly reveals the lasing action with several narrow peaks. The insets show the bright-field optical image and emission photograph of a single microplate with excitation above the lasing threshold, indicating that the standing waves of certain resonance frequencies are produced by reflecting the confined light multiple times at the end facets. As shown in Fig. 4(b), a transition from the linear region to the nonlinear region is displayed by the log–log plot of integrated PL intensity as a function of pump fluence, indicating the transformation of emission spectra from spontaneous emission to lasing action. In detail, by increasing the excitation fluence, the output intensity increases super-linearly and the FWHM of the lasing spectrum shrinks to 0.15 nm at pump intensity of . Furthermore, the mode competition is illustrated clearly from the set of sharp lasing peaks due to the mode selectively amplified by the optical positive feedback. According to the expression of quality factor of resonant cavity [44], where is the peak center wavelength and is the FWHM of the lasing spectrum, a typical emission peak is extracted to show the ultrahigh quality factor of 3551 with an FWHM of 0.15 nm in Fig. 4(c), which is among the best reported upconversion perovskite nanoplate lasers. Notably, in contrast to the distinct blueshift () of the lasing peak position from all-inorganic nanowire, nanorod, nanocuboid, and nanoplate [9,12,14,24], the lasing peak of only displays a slight fluctuation with less than 0.1 nm as shown in Fig. 10 in Appendix A.5 due to a compensation effect between the band-gap renormalization and Burstein–Moss effect [45], which proves the highly stable chromaticity of .
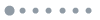
Figure 4.F-P mode lasing characterization of a single microplate. (a) Pump-intensity-dependent emission spectra under two-photon excitation. Inset: the bright-field optical image and emission photograph from the single microplate above the lasing threshold, indicating the F-P mode. The scale bar is 5 μm. (b) Output intensity (blue) and FWHM (red) of laser with increasing pump fluence. (c) Fitting of the lasing oscillation mode. The FWHM is 0.15 nm with a quality factor of .
To better match the purpose of the miniaturized device, it is more desirable to pursue a single-mode laser. Then we turn to investigating the WGM lasing of a microplate. When the excitation beam is focused on the corner of the microplate, the significantly strong emission at the corners confirms the WGM lasing, which is clearly observed in the inset of Fig. 5(a). Figure 11 in Appendix A.6 shows the total tested lasing spectra corresponding to the pump intensity. Selected spectra versus pump intensities are recorded in Fig. 5(a), displaying the evolution of emission spectra of with only one mode to be amplified in the largest gain region. Similar to the F-P mode lasing, the changed output intensities indicate the transformation of emission spectra from spontaneous emission to lasing action with an obvious inflection of emission intensity at in Fig. 5(b). Simultaneously, the FWHM rapidly narrows down to . To further evaluate the cavity performance, the quality factor is extracted as 3374 from the lasing FWHM of in Fig. 5(c). Moreover, the lasing peak position also only holds a slight shift from 0.15 nm as shown in Fig. 12 in Appendix A.7. In the WGM cavity, the optical losses are inevitable due to the field leakage from the cavity facet and edge scattering, indicating that the resonant wavelength could only exist in the highest gain region. The other modes experience small gain, which could be damped due to the loss, resulting in one remaining pattern. Furthermore, different from the lasing wavelength in the F-P cavity, the lasing wavelength in the WGM cavity shows an obvious redshift, which could be ascribed to the mode selection and intrinsic self-absorption arising from different laser cavities [46].
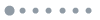
Figure 5.WGM lasing characterization of a single microplate. (a) Pump-intensity-dependent emission spectra under two-photon excitation. Inset: the bright-field optical image and the emission photograph from the single microplate above the lasing threshold, indicating the WGM lasing. The scale bar is 5 μm. (b) Output intensity (red) and FWHM (blue) of laser with increasing pump fluence. (c) Fitting of the lasing oscillation mode. The FWHM is 0.16 nm with a quality factor of .
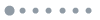
Figure 6.Log–log plot of the integrated PL intensity as a function of the pump fluence under two-photon excitation.
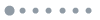
Figure 7.Pump-intensity-dependent SE dynamics.
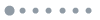
Figure 8.Normalized integrated intensity for microplates under pulsed excitation.
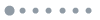
Figure 9.Two-photon pumped intensity-dependent emission spectra from a single microplate in the F-P cavity.
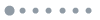
Figure 10.Center lasing position of the F-P mode laser.
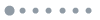
Figure 11.Two-photon pumped intensity-dependent emission spectra from a single microplate in the WGM cavity.
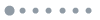
Figure 12.Center lasing position of the WGM laser.
Tailoring the lasing mode by the cavity size is a promising strategy for single-mode or multimode lasing. For a microplate, both the F-P mode oscillation between end facets and WGM oscillation among the four facets are possible, indicating that the optical mode could be modified as needed. Except for the microcavity effects due to the natural cavity structures, the high effective refractive index would result in excellent light confinement. Consequently, various resonance modes can be achieved. The distinct microcavity effects with mode selection would help us to fabricate functional miniaturized lasers by cavity structures, which will offer a versatile platform for the novel all-inorganic perovskites to be a flexible optical element into miniaturized photonic and optoelectronic devices.
4. CONCLUSION
In summary, we demonstrate the effective optical gain () with fast buildup in less than 1 ps from microplates for the first time to the best of our knowledge. Thanks to this, temperature-insensitive low-threshold ASE actions under two-photon excitation are observed from 100 K to 300 K with a high characteristic temperature of 403 K. Notably, two kinds of upconversion lasing, i.e., F-P mode with multimode lasing and WGM with single-mode lasing, are successfully realized at room temperature. The weak color shift of the lasing peak also confirms the strong temperature tolerance for heat induced by a pump laser with more stable chromaticity than the all-inorganic ones. The obtained ultrahigh quality factor () is among the best reported upconversion perovskite microplate lasers. Our findings suggest that the facile solution process ability and high performance lasing from a microplate will hold new opportunities in upconversion integrated photonic and mode selection optoelectronic devices for future exploration.
APPENDIX A
References
[1] R. M. Ma, R. F. Oulton. Applications of nanolasers. Nat. Nanotechnol., 14, 12-22(2019).
[2] B. Piccione, C. H. Cho, L. K. van Vugt, R. Agarwal. All-optical active switching in individual semiconductor nanowires. Nat. Nanotechnol., 7, 640-645(2012).
[3] M. T. Hill, M. C. Gather. Advances in small lasers. Nat. Photonics, 8, 908-918(2014).
[4] Q. Zhang, R. Su, W. Du, X. Liu, L. Zhao, S. T. Ha, Q. Xiong. Advances in small perovskite-based lasers. Small Methods, 1, 1700163(2017).
[5] L. N. Quan, B. P. Rand, R. H. Friend, S. G. Mhaisalkar, T. W. Lee, E. H. Sargent. Perovskites for next-generation optical sources. Chem. Rev., 119, 7444-7477(2019).
[6] K. Wang, S. Wang, S. Xiao, Q. Song. Recent advances in perovskite micro- and nanolasers. Adv. Opt. Mater., 6, 1800278(2018).
[7] J. Li, C. Ren, X. Qiu, X. Lin, R. Chen, C. Yin, T. He. Ultrafast optical nonlinearity of blue-emitting perovskite nanocrystals. Photon. Res., 6, 554-559(2018).
[8] C.-H. Lin, A. Verma, C.-Y. Kang, Y.-M. Pai, T.-Y. Chen, J.-J. Yang, C.-W. Sher, Y.-Z. Yang, P.-T. Lee, C.-C. Lin, Y.-C. Wu, S. K. Sharma, T. Wu, S.-R. Chung, H.-C. Kuo. Hybrid-type white LEDs based on inorganic halide perovskite QDs: candidates for wide color gamut display backlights. Photon. Res., 7, 579-585(2019).
[9] S. W. Eaton, M. Lai, N. A. Gibson, A. B. Wong, L. Dou, J. Ma, L. W. Wang, S. R. Leone, P. Yang. Lasing in robust cesium lead halide perovskite nanowires. Proc. Natl. Acad. Sci. USA, 113, 1993-1998(2016).
[10] H. Zhu, Y. Fu, F. Meng, X. Wu, Z. Gong, Q. Ding, M. V. Gustafsson, M. T. Trinh, S. Jin, X. Y. Zhu. Lead halide perovskite nanowire lasers with low lasing thresholds and high quality factors. Nat. Mater., 14, 636-642(2015).
[11] H. Zhou, S. Yuan, X. Wang, T. Xu, X. Wang, H. Li, W. Zheng, P. Fan, Y. Li, L. Sun, A. Pan. Vapor growth and tunable lasing of band gap engineered cesium lead halide perovskite micro/nanorods with triangular cross section. ACS Nano, 11, 1189-1195(2017).
[12] Z. Liu, J. Yang, J. Du, Z. Hu, T. Shi, Z. Zhang, Y. Liu, X. Tang, Y. Leng, R. Li. Robust subwavelength single-mode perovskite nanocuboid laser. ACS Nano, 12, 5923-5931(2018).
[13] B. Zhou, M. Jiang, H. Dong, W. Zheng, Y. Huang, J. Han, A. Pan, L. Zhang. High-temperature upconverted single-mode lasing in 3D fully inorganic perovskite microcubic cavity. ACS Photon., 6, 793-801(2019).
[14] Q. Zhang, R. Su, X. Liu, J. Xing, T. C. Sum, Q. Xiong. High-quality whispering-gallery-mode lasing from cesium lead halide perovskite nanoplatelets. Adv. Funct. Mater., 26, 6238-6245(2016).
[15] J. Yang, Z. Liu, F. Zeng, M. Pi, T. Shi, Y. Bian, X. Tang, J. Du, W. Liu, Y. Leng. High-quality single-mode lasers based on zero-dimensional cesium lead halide perovskites. Solar RRL, 3, 1900127(2019).
[16] B. Tang, H. Dong, L. Sun, W. Zheng, Q. Wang, F. Sun, X. Jiang, A. Pan, L. Zhang. Single-mode lasers based on cesium lead halide perovskite submicron spheres. ACS Nano, 11, 10681-10688(2017).
[17] Y. Wang, X. Li, X. Zhao, L. Xiao, H. Zeng, H. Sun. Nonlinear absorption and low-threshold multiphoton pumped stimulated emission from all-inorganic perovskite nanocrystals. Nano Lett., 16, 448-453(2016).
[18] L. Li, J. Ge, H. Wu, Q. H. Xu, S. Q. Yao. Organelle-specific detection of phosphatase activities with two-photon fluorogenic probes in cells and tissues. J. Am. Chem. Soc., 134, 12157-12167(2012).
[19] Y. Wang, V. D. Ta, Y. Gao, T. C. He, R. Chen, E. Mutlugun, H. V. Demir, H. D. Sun. Stimulated emission and lasing from CdSe/CdS/ZnS core-multi-shell quantum dots by simultaneous three-photon absorption. Adv. Mater., 26, 2954-2961(2014).
[20] Y. Xu, Q. Chen, C. Zhang, R. Wang, H. Wu, X. Zhang, G. Xing, W. W. Yu, X. Wang, Y. Zhang, M. Xiao. Two-photon-pumped perovskite semiconductor nanocrystal lasers. J. Am. Chem. Soc., 138, 3761-3768(2016).
[21] X. Li, W. Liu, Y. Song, H. Long, K. Wang, B. Wang, P. Lu. Two-photon-pumped high-quality, single-mode vertical cavity lasing based on perovskite monocrystalline films. Nano Energy, 68, 104334(2020).
[22] Y. Wang, X. Li, V. Nalla, H. Zeng, H. Sun. Solution-processed low threshold vertical cavity surface emitting lasers from all-inorganic perovskite nanocrystals. Adv. Funct. Mater., 27, 1605088(2017).
[23] Z. Liu, Z. Hu, Z. Zhang, J. Du, J. Yang, X. Tang, W. Liu, Y. Leng. Two-photon pumped amplified spontaneous emission and lasing from formamidinium lead bromine nanocrystals. ACS Photon., 6, 3150-3158(2019).
[24] X. Wang, H. Zhou, S. Yuan, W. Zheng, Y. Jiang, X. Zhuang, H. Liu, Q. Zhang, X. Zhu, X. Wang, A. Pan. Cesium lead halide perovskite triangular nanorods as high-gain medium and effective cavities for multiphoton-pumped lasing. Nano Res., 10, 3385-3395(2017).
[25] Z. Gu, K. Wang, W. Sun, J. Li, S. Liu, Q. Song, S. Xiao. Two-photon pumped CH3NH3PbBr3 perovskite microwire lasers. Adv. Opt. Mater., 4, 472-479(2016).
[26] Q. Wei, B. Du, B. Wu, J. Guo, M. J. Li, J. Fu, Z. Zhang, J. Yu, T. Hou, G. Xing, T. C. Sum, W. Huang. Two-photon optical properties in individual organic-inorganic perovskite microplates. Adv. Opt. Mater., 5, 1700809(2017).
[27] C. Huang, K. Wang, Z. Yang, L. Jiang, R. Liu, R. Su, Z.-K. Zhou, X. Wang. Up-conversion perovskite nanolaser with single mode and low threshold. J. Phys. Chem. C, 121, 10071-10077(2017).
[28] X. He, P. Liu, H. Zhang, Q. Liao, J. Yao, H. Fu. Patterning multicolored microdisk laser arrays of cesium lead halide perovskite. Adv. Mater., 29, 1604510(2017).
[29] W. Zhang, L. Peng, J. Liu, A. Tang, J. S. Hu, J. Yao, Y. S. Zhao. Controlling the cavity structures of two-photon-pumped perovskite microlasers. Adv. Mater., 28, 4040-4046(2016).
[30] J. Xu, X. Li, J. Xiong, C. Yuan, S. Semin, T. Rasing, X. H. Bu. Halide perovskites for nonlinear optics. Adv. Mater., 32, 1806736(2020).
[31] S. Wang, K. Wang, Z. Gu, Y. Wang, C. Huang, N. Yi, S. Xiao, Q. Song. Solution-phase synthesis of cesium lead halide perovskite microrods for high-quality microlasers and photodetectors. Adv. Opt. Mater., 5, 1700023(2017).
[32] X. Tang, Z. Hu, W. Yuan, W. Hu, H. Shao, D. Han, J. Zheng, J. Hao, Z. Zang, J. Du, Y. Leng, L. Fang, M. Zhou. Perovskite CsPb2Br5 microplate laser with enhanced stability and tunable properties. Adv. Opt. Mater., 5, 1600788(2017).
[33] C. Han, C. Li, Z. Zang, M. Wang, K. Sun, X. Tang, J. Du. Tunable luminescent CsPb2Br5 nanoplatelets: applications in light-emitting diodes and photodetectors. Photon. Res., 5, 473-480(2017).
[34] G. Tong, H. Li, D. Li, Z. Zhu, E. Xu, G. Li, L. Yu, J. Xu, Y. Jiang. Dual-phase CsPbBr3-CsPb2Br5 perovskite thin films via vapor deposition for high-performance rigid and flexible photodetectors. Small, 14, 1702523(2018).
[35] X. Zhang, Z. Jin, J. Zhang, D. Bai, H. Bian, K. Wang, J. Sun, Q. Wang, S. F. Liu. All-ambient processed binary CsPbBr3-CsPb2Br5 perovskites with synergistic enhancement for high-efficiency Cs-Pb-Br-based solar cells. ACS Appl. Mater. Interfaces, 10, 7145-7154(2018).
[36] Z. Xiao, W. Meng, J. Wang, D. B. Mitzi, Y. Yan. Searching for promising new perovskite-based photovoltaic absorbers: the importance of electronic dimensionality. Mater. Horiz., 4, 206-216(2017).
[37] B. R. Sutherland, E. H. Sargent. Perovskite photonic sources. Nat. Photonics, 10, 295-302(2016).
[38] K.-H. Wang, L. Wu, L. Li, H.-B. Yao, H.-S. Qian, S.-H. Yu. Large-scale synthesis of highly luminescent perovskite-related CsPb2Br5 nanoplatelets and their fast anion exchange. Angew. Chem. (Int. Ed.), 55, 8328-8332(2016).
[39] S. De Wolf, J. Holovsky, S. J. Moon, P. Loper, B. Niesen, M. Ledinsky, F. J. Haug, J. H. Yum, C. Ballif. Organometallic halide perovskites: sharp optical absorption edge and its relation to photovoltaic performance. J. Phys. Chem. Lett., 5, 1035-1039(2014).
[40] P. Geiregat, J. Maes, K. Chen, E. Drijvers, J. De Roo, J. M. Hodgkiss, Z. Hens. Using bulk-like nanocrystals to probe intrinsic optical gain characteristics of inorganic lead halide perovskites. ACS Nano, 12, 10178-10188(2018).
[41] J. Fu, Q. Xu, G. Han, B. Wu, C. H. A. Huan, M. L. Leek, T. C. Sum. Hot carrier cooling mechanisms in halide perovskites. Nat. Commun., 8, 1300(2017).
[42] A. Ohtomo, K. Tamura, M. Kawasaki, T. Makino, Y. Segawa, Z. K. Tang, G. K. L. Wong, Y. Matsumoto, H. Koinuma. Room-temperature stimulated emission of excitons in ZnO/(Mg, Zn)O superlattices. Appl. Phys. Lett., 77, 2204-2206(2000).
[43] J. J. Coleman, J. D. Young, A. Garg. Semiconductor quantum dot lasers: a tutorial. J. Lightwave Technol., 29, 499-510(2011).
[44] X. Zhuang, Y. Ouyang, X. Wang, A. Pan. Multicolor semiconductor lasers. Adv. Opt. Mater., 7, 1900071(2019).
[45] J. C. Johnson, H. J. Choi, K. P. Knutsen, R. D. Schaller, P. Yang, R. J. Saykally. Single gallium nitride nanowire lasers. Nat. Mater., 1, 106-110(2002).
[46] X. Liu, Q. Zhang, Q. Xiong, T. C. Sum. Tailoring the lasing modes in semiconductor nanowire cavities using intrinsic self-absorption. Nano Lett., 13, 1080-1085(2013).