Alexander I. Chernov1、2、*, Mikhail A. Kozhaev1、2, Anastasiia Khramova1、3, Alexander N. Shaposhnikov4, Anatoly R. Prokopov4, Vladimir N. Berzhansky4, Anatoly K. Zvezdin1、2, and Vladimir I. Belotelov1、3
Author Affiliations
1Russian Quantum Center, 45, Skolkovskoye shosse, Moscow 121353, Russia2Prokhorov General Physics Institute of the Russian Academy of Sciences, 38 Vavilov Street, Moscow 119991, Russia3Faculty of Physics, Lomonosov Moscow State University, Leninskie Gory, Moscow 119991, Russia4Vernadsky Crimean Federal University, 4 Vernadskogo Prospekt, Simferopol 295007, Russiashow less
Abstract
The inverse Faraday effect induced in magnetic films by ultrashort laser pulses allows excitation and control of spins at gigahertz and sub-terahertz frequencies. The frequency of the optically excited magnetization precession is easily tunable by the external magnetic field. On the other hand, the initial phase of the precession marginally depends on the magnetic field. Here we demonstrate an approach for the control of the precession phase by variation of the pump beam direction. In particular, we consider the case when the magnetization precession is excited by obliquely incident pump pulses in a magnetic dielectric film placed in the in-plane magnetic field. Theoretical consideration predicts that the initial phase should appear for a non-zero in-plane component of the pump wavevector orthogonal to the external magnetic field. Experimental studies confirm this conclusion and reveal that the phase grows with increase of the in-plane wavevector component. Variation of phase by 15 deg is demonstrated. Potentially, the phase could be changed even more pronouncedly by more than 90 deg. This work provides a simple way for additional manipulation with optically excited magnetization dynamics, which is of importance for different spintronic applications.1. INTRODUCTION
All-optical excitation and detection of spin dynamics by ultrashort laser pulses have opened new ways for manipulation and control of spins at femtosecond and picosecond time scales [1–6]. In the case of transparent magnetic films, the laser pulse influences the magnetization of the sample non-thermally by either the inverse Faraday effect [7–9] or by photoinduced magnetocrystalline anisotropy [8,10,11]. The former is observed for circularly polarized pulses and can be interpreted in terms of the effective magnetic field induced within the sample during pulse propagation. In contrast, modifications of magnetocrystalline anisotropy appear for linearly polarized pulses.
The optical approach to excitation of the magnetization dynamics overcomes several limitations inherent for the conventional methods utilizing microwaves. First of all, it allows influence on the magnetization locally, within the focused laser spot, that can be easily shifted along the sample [12,13]. This feature can be used to study spin wave propagation in nanostructured materials with high resolution [14]. The wave properties of light make it possible to control sample magnetization in a certain region of a magnet layer within a micrometer by multiple excitation pulses [15]. The micrometer size area of the sample where spins interact with photons can operate like a point source of magnons [16–22]. Subwavelength localization of the magnon source along the magnetic film thickness has recently been demonstrated [23]. Variation of the laser spot shape and size provides tunability in terms of the type and spectrum of the generated spin waves. For example, one could switch between surface and backward volume spin waves by simply reducing the diameter of the laser spot [19,20]. Moreover, passing from excitation with a single pulse to multiple pulse excitation gives an additional degree of freedom leading to enhancement of the spin wave amplitude, tunability of their spectrum, and directivity of the magnon source [17,18].
Usually, optically excited magnetization dynamics is observed in the pump–probe experiment in which a high-intensity pump beam drives spins and a low-intensity probe beam arrives at some time delay and measures variation of the magnetization along its wavevector by the Faraday effect. The magnetization precession at a given point is described by a decaying harmonic function, , where the axis is perpendicular to the precession axis, is the projection of the magnetization on the axis, , , and are precession amplitude, frequency, and phase, respectively, and is the decay time. The precession amplitude is increased by raising the excitation energy fluence, while the frequency is changed via the external magnetic field. At the same time, control of the phase is not so straightforward. If magnetization dynamics is excited through the photoinduced magnetic anisotropy or inverse Cotton–Mouton effect, then the phase can be modified by orientation of the linear polarization of the pumping beam [11,22]. However, in the case of the inverse Faraday effect dealing with circularly polarized laser pulses, only two scenarios are available: clockwise and anti-clockwise magnetization precession excited by left and right circularly polarized pulses. This corresponds to and . Thus, tunable adjustment of the phase has not yet been demonstrated.
Sign up for Photonics Research TOC. Get the latest issue of Photonics Research delivered right to you!Sign up now
In this work we demonstrate the approach for the precise variation of the magnetization oscillation phase excited by circularly polarized femtosecond laser pulses. Theoretical investigation reveals that the phase depends on the direction of the obliquely incident pump pulses. The experimental studies confirm this behavior and are in good agreement with the theoretical model. The obtained results open a new way for the precise modification of the phase of the magnetization dynamics and, in particular, spin waves excited by circularly polarized light pulses.
2. PHASE OF THE MAGNETIZATION PRECESSION EXCITED VIA THE INVERSE FARADAY EFFECT
We start with the theoretical consideration of the magnetization dynamics excited within the illuminated spot of the film. Figure 1 shows the considered system geometry, where the circularly polarized pump beam enters the material at some incidence angle. It induces the effective magnetic field directed along the wavevector of light inside the magnetic film. Consequently, the orientation of is defined by the refraction angle of the pump and its azimuth angle . The field exists in the illuminated area of the magnetic film during the pulse propagation through the sample. Because of the pulse of , magnetization of the sample becomes locally nudged and magnetization oscillations spread away in the form of spin waves. Therefore, generally, the magnetization dynamics should be described by the function . However, the main properties of the optically induced magnetization dynamics within the illuminated spot can be considered in a simplified model that assumes a uniform precession of the magnetization . In this model, generation of spin waves can be taken into account by effective damping parameter that exceeds Gilbert parameter .
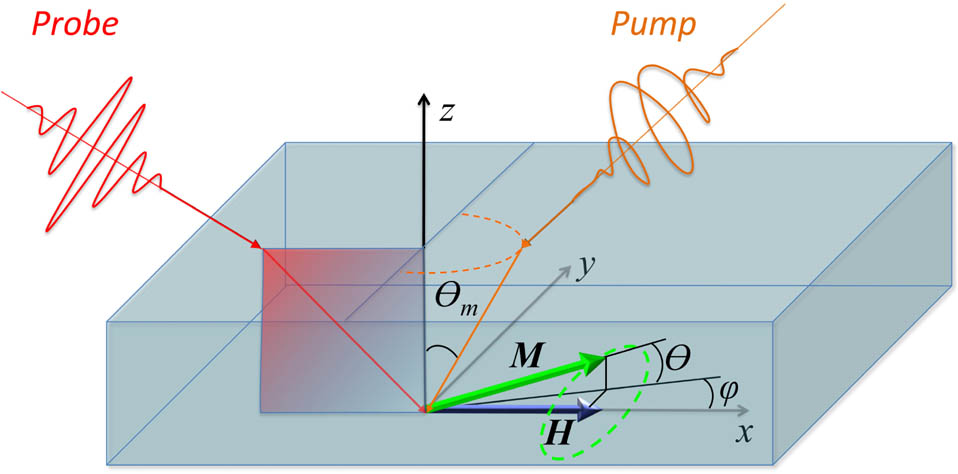
Figure 1.Configuration of the sample illumination with the pump and probe laser pulses. is the external magnetic field. Before optical excitation, magnetization is directed along . A circularly polarized pump beam induces the magnetization dynamics, resulting in precession along a trajectory that is depicted by the green dashed line.
The magnetization dynamics is governed by the Landau–Lifshitz–Gilbert equation. In the spherical coordinate system with the axis along the normal to the film and the axis along the in-plane external magnetic field , it is written by Here, is the polar angle of magnetization, is the azimuth angle of magnetization (Fig. 1), is the gyromagnetic ratio, and is the free energy density of the magnetic film. In the case of the predominant growth anisotropy with respect to the crystalline one, the free energy density of the magnetic film is written by where is a constant of the uniaxial magnetic anisotropy. The first term in Eq. (2) describes the magnetic anisotropy caused by the planar shape of the magnetic sample and its growth. The magnetic anisotropy can be characterized by the effective anisotropy field . In the absence of , the magnetization lies in-plane, which gives the equilibrium state and . Passing to , linearizing Eq. (1) and taking Eq. (2) into account leads to the following set of equations: where and . It follows from these equations that input of in the excitation of the magnetization is negligibly small with respect to the input of the other components. The problem of solving a set of linear non-homogeneous differential equations [Eqs. (3)] with ultrashort, -function-like pulses can be reduced to the problem of solving a set of homogeneous equations with initial conditions where are amplitudes of the IFE field pulses, , and it is assumed that .
Since is parallel to the -wavevector of light inside the magnetic film, then (Fig. 1), where and are polar and azimuth angles of inside the magnetic film. The magnetization dynamics excited by the laser pulse is determined by solution of Eq. (3) for and is described by the free magnetization precession given by where Expressions for the amplitude and phase are obtained in view of Eq. (4). Generally, they are rather cumbersome, but for can be notably simplified: Therefore, one can see that the initial phase of the magnetization precession appears if the in-plane component of orthogonal to the external magnetic field () is non-vanishing. For relatively small (), the phase increases linearly with . For magnetic films with in-plane uniaxial magnetic anisotropy and in small magnetic fields, the ratio of might be rather large, which provides notable variation of the phase even for moderate incidence angles.
3. EXPERIMENT
For the experimental investigation we have used rare-earth iron-garnet film with bismuth ion substitution () [24]. It was grown by liquid phase epitaxy on a gadolinium gallium garnet substrate with a crystallographic orientation (111). The film thickness is 4.1 μm, the saturation magnetization is , the uniaxial anisotropy constant is negative and equals , while is .
The sample is studied by the two-color pump–probe technique. The pump wavelength is 616 nm, and pump pulses are circularly polarized and cause the magnetization precession. The light energy fluence is (calculated for 9 μm beam diameter). The probe pulse wavelength is 820 nm and has 15 times less energy fluence. Both pump and probe 150 fs pulses are generated by a Newport Mai Tai HP Ti:sapphire laser and a Spectra-Physics Inspire Auto 100 optical parametric oscillator at a 80.54 MHz repetition rate. The Faraday effect for the transmitted probe pulses is used for detection of the magnetization precession (Nirvana balanced diode detector with lock-in amplifier). The time delay between the pump and probe pulses is varied from to 2.8 ns, where zero-time delay corresponds to the simultaneous propagation of the pump and probe pulses through the sample.
Focusing of the pump and probe beams is performed by the single reflective microscope objective. Direction of the probe beam is fixed in the x–z plane at 17 deg incidence [Fig. 2(a)]. In contrast, the azimuth angle of the pump beam varies from to . The incidence angle of the pump is .
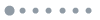
Figure 2.(a) Scheme of the experiment with the variation of the pump beam position with changing from up to . (b) Normalized time-resolved change of the Faraday rotation indicating the magnetization precession excited by laser pulses in different external magnetic fields from 15 to 850 Oe. Inset: dependence of the precession frequency on the external magnetic field.
4. DEPENDENCE OF THE PRECESSION PHASE ON THE PUMP AZIMUTH ANGLE
The magnetization precession excited by laser pulses in different external magnetic fields from is shown in Fig. 2(b). Its temporal dependence is well described with the decaying harmonic function by Eq. (5). The decay time of the magnetization precession in is , which, in accordance with Eq. (7), corresponds to the damping parameter . The oscillation frequency grows almost linearly with the magnetic field [inset in Fig. 2(b)]. It is also in agreement with theory, in particular with Eq. (6). Some deviation from Eq. (6) appears for relatively small magnetic fields, , which can be accounted for by excitation of the spin waves and for some inhomogeneity of the sample.
Let us now vary the orientation of the pump beam as per the scheme in Fig. 2(a) and pay attention to the initial phase of the excited magnetization precession [Fig. 3(a)]. One can note the trend of the phase change with variation of the azimuth angle. If the observed magnetization precession curves are fitted by Eq. (5), then the phase dependence on the incidence angle is quantified [red circles in Fig. 3(b)]. The experimentally obtained data is well described by Eq. (9) in the limit of if some additive term is introduced: [blue line in Fig. 3(b)]. The presence of the term can be due to some inaccuracy in the determination of the angles and and to the influence of the thermal effects, which are not accounted for in the theory above. Nevertheless, the line slope is close to the reciprocal of the refractive index of the magnetic film , which agrees with Eq. (9). Indeed, for a relatively small incidence angle and large magnetic fields, where the oscillation frequency is linear in , the ratio of and .
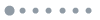
Figure 3.(a) Normalized time-resolved change of the Faraday rotation indicating magnetization precession excited by pump beams of different azimuth angles. The pump beam azimuth angle varies from to . Inset: zoom for easier tracking of the phase change. The black line is a guide for the eye demonstrating the A peak position change. All curves have offsets for clarity of representation. (b) Dependence of the phase angle on the sine of the pump azimuth angle. The angle of pump incidence is 17 deg. The external magnetic field is 850 Oe.
Change of the initial phase with variation of the azimuth angle can be increased if is much larger than . This might be possible for films with large magnetic anisotropy. Thus, a film with Gs and in the external magnetic field will have , which implies for the incidence angle of .
5. CONCLUSION
In conclusion, we have demonstrated the approach for phase shift of the magnetization oscillations and spin waves optically excited by circularly polarized laser pulses. It enables the precise control of the magnetization dynamics parameters for the case when the inverse Faraday effect is responsible for its excitation. The experimental data is in good agreement with the proposed theoretical interpretation of the effect, rendering the linear trend for the phase angle change with the variation of the pump light azimuth angle. We demonstrate a 15 deg phase shift obtained with rotation of the pump beam plane of incidence by 180 deg at a fixed incidence angle of 17 deg. The experimental sample film is not optimized for this effect. Calculations predict that the oscillation phase could be varied by almost 90 deg in the case when a magnetic film of high in-plane magnetic anisotropy is placed in a relatively small external magnetic field of a few tens of Oe and is illuminated at a large incidence angle. This study provides a simple and effective way for precise manipulation of ultrafast magnetization dynamics, which is of increasing importance for spintronic and magnetophotonic applications.
References
[1] A. V. Kimel, A. Kirilyuk, P. A. Usachev, R. V. Pisarev, A. M. Balbashov, T. Rasing. Ultrafast non-thermal control of magnetization by instantaneous photomagnetic pulses. Nature, 435, 655-657(2005).
[2] E. Beaurepaire, J. C. Merle, A. Daunois, J. Y. Bigot. Ultrafast spin dynamics in ferromagnetic nickel. Phys. Rev. Lett., 76, 4250-4253(1996).
[3] J.-Y. Bigot, M. Vomir, E. Beaurepaire. Coherent ultrafast magnetism induced by femtosecond laser pulses. Nat. Phys., 5, 515-520(2009).
[4] A. Stupakiewicz, K. Szerenos, D. Afanasiev, A. Kirilyuk, A. V. Kimel. Ultrafast nonthermal photo-magnetic recording in a transparent medium. Nature, 542, 71-74(2017).
[5] D. Bossini, V. I. Belotelov, A. K. Zvezdin, A. N. Kalish, A. V. Kimel. Magnetoplasmonics and femtosecond optomagnetism at the nanoscale. ACS Photon., 3, 1385-1400(2016).
[6] P. Němec, M. Fiebig, T. Kampfrath, A. V. Kimel. Antiferromagnetic opto-spintronics. Nat. Phys., 14, 229-241(2018).
[7] L. P. Pitaevskii. Electric forces in a transparent dispersive medium. J. Exp. Theor. Phys., 39, 1450-1458(1960).
[8] F. Hansteen, A. Kimel, A. Kirilyuk, T. Rasing. Nonthermal ultrafast optical control of the magnetization in garnet films. Phys. Rev. B, 73, 014421(2006).
[9] R. V. Mikhaylovskiy, E. Hendry, V. V. Kruglyak. Ultrafast inverse Faraday effect in a paramagnetic terbium gallium garnet crystal. Phys. Rev. B, 86, 100405(2012).
[10] F. Atoneche, A. M. Kalashnikova, A. V. Kimel, A. Stupakiewicz, A. Maziewski, A. Kirilyuk, T. Rasing. Large ultrafast photoinduced magnetic anisotropy in a cobalt-substituted yttrium iron garnet. Phys. Rev. B, 81, 214440(2010).
[11] L. A. Shelukhin, V. V. Pavlov, P. A. Usachev, P. Y. Shamray, R. V. Pisarev, A. M. Kalashnikova. Ultrafast laser-induced changes of the magnetic anisotropy in a low-symmetry iron garnet film. Phys. Rev. B, 97, 014422(2018).
[12] C. D. Stanciu, F. Hansteen, A. V. Kimel, A. Kirilyuk, A. Tsukamoto, A. Itoh, T. Rasing. All-optical magnetic recording with circularly polarized light. Phys. Rev. Lett., 99, 047601(2007).
[13] A. I. Chernov, M. A. Kozhaev, P. M. Vetoshko, D. V. Dodonov, A. R. Prokopov, A. G. Shumilov, A. N. Shaposhnikov, V. N. Berzhanskii, A. K. Zvezdin, V. I. Belotelov. Local probing of magnetic films by optical excitation of magnetostatic waves. Phys. Solid State, 58, 1128-1134(2016).
[14] A. V. Sadovnikov, C. S. Davies, V. V. Kruglyak, D. V. Romanenko, S. V. Grishin, E. N. Beginin, Y. P. Sharaevskii, S. A. Nikitov. Spin wave propagation in a uniformly biased curved magnonic waveguide. Phys. Rev. B, 96, 060401(2017).
[15] C. S. Davies, J. Janušonis, A. V. Kimel, A. Kirilyuk, A. Tsukamoto, T. Rasing, R. I. Tobey. Towards massively parallelized all-optical magnetic recording. J. Appl. Phys., 123, 213904(2018).
[16] T. Satoh, Y. Terui, R. Moriya, B. A. Ivanov, K. Ando, E. Saitoh, T. Shimura, K. Kuroda. Directional control of spin-wave emission by spatially shaped light. Nat. Photonics, 6, 662-666(2012).
[17] M. Jäckl, V. I. Belotelov, I. A. Akimov, I. V. Savochkin, D. R. Yakovlev, A. K. Zvezdin, M. Bayer. Magnon accumulation by clocked laser excitation as source of long-range spin waves in transparent magnetic films. Phys. Rev. X, 7, 021009(2017).
[18] I. V. Savochkin, M. Jäckl, V. I. Belotelov, I. A. Akimov, M. A. Kozhaev, D. A. Sylgacheva, A. I. Chernov, A. N. Shaposhnikov, A. R. Prokopov, V. N. Berzhansky, D. R. Yakovlev, A. K. Zvezdin, M. Bayer. Generation of spin waves by a train of fs-laser pulses: a novel approach for tuning magnon wavelength. Sci. Rep., 7, 5668(2017).
[19] A. I. Chernov, M. A. Kozhaev, I. V. Savochkin, D. V. Dodonov, P. M. Vetoshko, A. K. Zvezdin, V. I. Belotelov. Optical excitation of spin waves in epitaxial iron garnet films: MSSW vs BVMSW. Opt. Lett., 42, 279-282(2017).
[20] A. I. Chernov, M. A. Kozhaev, A. K. Zvezdin, V. I. Belotelov. Generation of spin waves by fs-laser pulses in transparent magnetic films: role of the laser beam diameter. J. Phys., 869, 012020(2017).
[21] I. Yoshimine, Y. Y. Tanaka, T. Shimura, T. Satoh. Unidirectional control of optically induced spin waves. Europhys. Lett., 117, 67001(2017).
[22] I. Yoshimine, T. Satoh, R. Iida, A. Stupakiewicz, A. Maziewski, T. Shimura. Phase-controllable spin wave generation in iron garnet by linearly polarized light pulses. J. Appl. Phys., 116, 043907(2014).
[23] M. A. Kozhaev, A. I. Chernov, D. A. Sylgacheva, A. N. Shaposhnikov, A. R. Prokopov, V. N. Berzhansky, A. K. Zvezdin, V. I. Belotelov. Giant peak of the inverse Faraday effect in the band gap of magnetophotonic microcavity. Sci. Rep., 8, 11435(2018).
[24] A. R. Prokopov, P. M. Vetoshko, A. G. Shumilov, A. N. Shaposhnikov, A. N. Kuz’michev, N. N. Koshlyakova, V. N. Berzhansky, A. K. Zvezdin, V. I. Belotelov. Epitaxial BiGdSc iron-garnet films for magnetophotonic applications. J. Alloys Compd., 671, 403-407(2016).