
- Chinese Optics Letters
- Vol. 20, Issue 7, 073701 (2022)
Abstract
1. Introduction
Terahertz (THz) radiation refers to electromagnetic waves ranging from 0.1 to 10 THz. The evolution of THz technology has inspired many innovative THz applications in various areas, including communication, imaging, and astronomy[
In 2014, a new method called laser-induced graphene (LIG) was proposed[
In this study, we propose a THz graphene grid absorber fabricated through the LIG method using a
Sign up for Chinese Optics Letters TOC. Get the latest issue of Chinese Optics Letters delivered right to you!Sign up now
2. Experimental Details
A schematic of the fabrication process is shown in Fig. 1(a). A
Figure 1.(a) Schematic of LIG fabrication. (b) Grid graphene THz absorber. (c) Unit cell of the absorber.
3. Results and Discussion
Figure 2(a) shows one of the fabricated samples. The microscopic image of the sample is shown in Fig. 2(b). The sample was induced with a laser power of 24 W and scanning speed of 230 mm/s. Thereafter, we used a Raman spectrometer to measure the Raman spectra of the samples. Figure 2(c) shows the Raman spectra of LIG and PI. The LIG Raman spectra depicted in Fig. 2(c) illustrate the D, G, and 2D peaks at 1359, 1583, and
Figure 2.(a) Sample of the LIG absorber. (b) Graphene grid observed under a microscope. (c) Raman spectra of the LIG and PI. (d) SEM image of the LIG. (e) SEM image of the cross section of the LIG.
To investigate the relationship between the performance of the absorber and laser power, the temporal spectra of the absorbers with different laser powers were measured using a THz time-domain spectroscopy system (THz-TDS, TAS7400SP; Advantest Corporation) with a transmission analysis accessory. We set the laser power to range from 40% to 80% of the maximal laser power (
Figure 3.(a) Time-domain results of the THz graphene grid absorbers induced by the different laser power ranging from 40% to 80%. (b) Corresponding transmittance spectra, (c) reflectance spectra, and (d) absorption spectra of the absorbers.
Next, the laser-scanning-speed-dependent morphology and THz absorption of the graphene grid absorbers were characterized. We changed the laser scanning speed from 190 mm/s to 270 mm/s when the laser power was fixed at 70% of
Figure 4.(a) Graphene grid absorbers induced with different laser scanning speeds under a microscope. (b) Corresponding experimental and simulated absorption spectra of the absorbers. (c) Simulated THz near-field distributions of the absorber (W = 300 µm) at a frequency of 0.8 THz, 1 THz, 1.2 THz, and 1.5 THz, respectively.
We obtained two broadband LIG grid absorbers with a good performance of 90% absorption from 0.5 THz to 2 THz. One was induced with a laser power of 70% and scanning speed of 190 mm/s, and the other was induced with a laser power of 80% and scanning speed of 230 mm/s. This can be proven from the laser flux
The laser flux is a key factor in THz absorption. When
4. Conclusion
A grid graphene broadband absorber for the THz range was proposed based on the LIG method. We investigated the influence of varying laser power and scanning speed on the absorber performance. It was found that we can coarsely tune the absorption by changing the laser power and subtly tune the absorption by changing the scanning speed. With optimized parameters, the fabricated absorber exhibited a high absorbing capacity of over 90% within a broad bandwidth ranging from 0.5 to 2 THz. Furthermore, the grid structure of our absorber makes the device more robust and flexible than an unpatterned LIG film. Through this easy and cost-effective LIG method, various carbon-based THz devices are expected, such as filters and modulators, which may have great potential in numerous applications, ranging from THz communication to THz imaging and sensing.
References
[1] J. Federici, L. Moeller. Review of terahertz and subterahertz wireless communications. J. Appl. Phys., 107, 111101(2010).
[2] M. Tonouchi. Cutting-edge terahertz technology. Nat. Photonics, 1, 97(2007).
[3] T. Nagatsuma, G. Ducournau, C. C. Renaud. Advances in terahertz communications accelerated by photonics. Nat. Photonics, 10, 371(2016).
[4] Y. Shen, Z. Shen, G. Zhao, W. Hu. Photopatterned liquid crystal mediated terahertz Bessel vortex beam generator. Chin. Opt. Lett., 18, 080003(2020).
[5] H. T. Chen, W. J. Padilla, M. J. Cich, A. K. Azad, R. D. Averitt, A. J. Taylor. A metamaterial solid-state terahertz phase modulator. Nat. Photonics, 3, 148(2009).
[6] H. Zhuang, C. Liu, F. Li, J. Zhuang, K. Li. Tunable plasmonic filter based on parallel bulk Dirac semimetals at terahertz frequencies. Appl. Opt., 60, 3634(2021).
[7] H. Sun, J. Yang, H. Liu, D. Wu, X. Zheng. Process-controllable modulation of plasmon-induced transparency in terahertz metamaterials. Chin. Opt. Lett., 19, 013602(2021).
[8] Y. Chen, J. Li, C. He, J. Qin, X. Chen, S. Li. Enhancement of high transmittance and broad bandwidth terahertz metamaterial filter. Opt. Mater., 115, 111029(2021).
[9] H. Deljoo, A. Rostami. Broadband terahertz absorber using superimposed graphene quantum dots. Opt. Quantum Electron., 53, 442(2021).
[10] J. Bai, Z. Pang, P. Shen, T. Chen, W. Shen, S. Wang, S. Chang. A terahertz photo-thermoelectric detector based on metamaterial absorber. Opt. Commun., 497, 127184(2021).
[11] Z. Shen, S. Li, Y. Xu, W. Yin, X. Chen. Three-dimensional printed ultrabroadband terahertz metamaterial absorbers. Phys. Rev. Appl., 16, 014066(2021).
[12] J. Xiao, R. Xiao, R. Zhang, Z. Shen, W. Hu, L. Wang, Y. Lu. Tunable terahertz absorber based on transparent and flexible metamaterial. Chin. Opt. Lett., 18, 092403(2020).
[13] M. Rahm, J. S. Li, W. J. Padilla. THz wave modulators: a brief review on different modulation techniques. J. Infrared Millim. Terahertz Waves, 34, 1(2012).
[14] K. D. Xu, J. Li, A. Zhang, Q. Chen. Tunable multi-band terahertz absorber using a single-layer square graphene ring structure with T-shaped graphene strips. Opt. Express, 28, 11482(2020).
[15] T. Hu, N. I. Landy, C. M. Bingham, X. Zhang, R. D. Averitt, W. J. Padilla. A metamaterial absorber for the terahertz regime: design, fabrication and characterization. Opt. Express, 16, 7181(2008).
[16] F. J. Bosquespadilla, L. N. Landy, W. K. Smith, J. D. Smith, E. Padilla, S. Sajuyigbe. Perfect metamaterial absorber. Phys. Rev. Lett., 100, 207402(2008).
[17] J. Huang, T. Fu, H. Li, Z. Shou, X. Gao. A reconfigurable terahertz polarization converter based on metal–graphene hybrid metasurface. Chin. Opt. Lett., 18, 013102(2020).
[18] X. Shen, Y. Yang, Y. Zang, J. Gu, J. Han, W. Zhang. Triple-band terahertz metamaterial absorber: design, experiment, and physical interpretation. Appl. Phys. Lett., 101, 207402(2012).
[19] J. Grant, Y. Ma, S. Saha, A. Khalid, D. R. Cumming. Polarization insensitive, broadband terahertz metamaterial absorber. Opt. Lett., 36, 3476(2011).
[20] J. Lin, Z. Peng, Y. Liu, F. Ruiz-Zepeda, R. Ye, E. L. G. Samuel, M. J. Yacaman, B. I. Yakobson, J. M. Tour. Laser-induced porous graphene films from commercial polymers. Nat. Commun., 5, 5714(2014).
[21] A. K. Geim, K. S. Novoselov. The rise of graphene. Nat. Mater., 6, 183(2007).
[22] W. Liu, J. Wei, X. Sun, H. Yu. A study on graphene—metal contact. Crystals, 3, 257(2013).
[23] M. G. Stanford, K. Yang, Y. Chyan, C. Kittrell, J. M. Tour. Laser induced graphene for flexible and embeddable gas sensors. ACS Nano, 13, 3474(2019).
[24] Z. Peng, J. Lin, R. Ye, E. Samuel, J. M. Tour. Flexible and stackable laser-induced graphene supercapacitors. ACS Appl. Mater. Interfaces, 7, 3414(2015).
[25] M. G. Stanford, J. T. Li, Y. Chyan, Z. Wang, J. M. Tour. Laser-induced graphene triboelectric nanogenerators. ACS Nano, 13, 7166(2019).
[26] Z. Wang, G. Wang, G. Liu, B. Hu, Y. Zhang. Patterned laser-induced graphene for terahertz wave modulation. J. Opt. Soc. Am. B, 37, 546(2020).
[27] R. Zhang, G. Zong, S. Wu, R. Song, X. Zhang, S. Ge, W. Hu, L. Wang, Y. Lu. Ultrathin flexible terahertz metamaterial bandstop filter based on laser-induced graphene. J. Opt. Soc. Am. B, 39, 1229(2022).
[28] Y. Chyan, R. Ye, Y. Li, S. P. Singh, C. J. Arnusch, J. M. Tour. Laser-induced graphene by multiple lasing: toward electronics on cloth, paper, and food. ACS Nano, 12, 2176(2018).
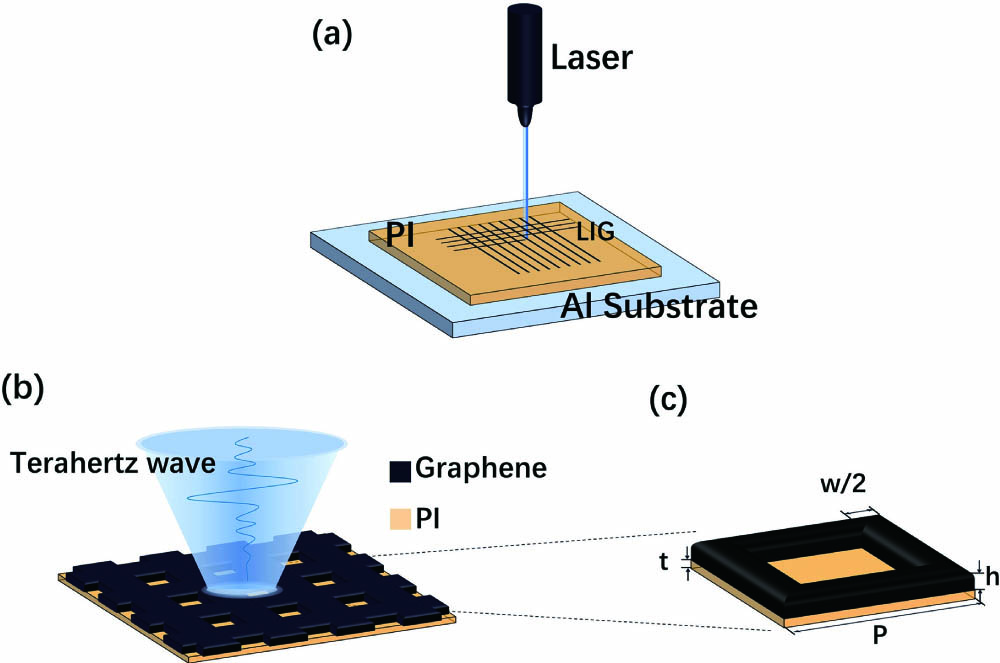
Set citation alerts for the article
Please enter your email address