Author Affiliations
1Shenzhen Key Laboratory of Laser Engineering, Key Laboratory of Advanced Optical Precision Manufacturing Technology of Guangdong Higher Education Institutes, College of Optoelectronic Engineering, Shenzhen University, Shenzhen 518060, China2Institute of Applied Physics and Materials Engineering, University of Macau, Macau, Chinashow less
Abstract
Employing 0.3 nm diameter single-walled carbon nanotubes (SWCNTs) as saturable absorbers, we demonstrate a passively mode-locked fiber laser operating at 1950 nm. The 0.3 nm diameter SWCNTs are prepared by pyrolyzing dipropylamine in the channels of zeolite crystals MgAPO-11 (AEL). The laser pumped by a 1550 nm laser source produces 972 fs pulses with a spectral width at half-maximum of 4.2 nm and a repetition rate of 21.05 MHz, an average output power of 2.3 mW corresponding to the maximum pump power of 420 mW with a 10% output coupler.Recently, pulsed lasers emitting at the eye-safe wavelength region of 2 μm have attracted a lot of attention due to their wide applications in medicine, spectroscopy, nonlinear optics, remote sensing, synchronously-pumped optical parametric oscillators, and so on[1–5]. In order to generate ultrashort 2 μm pulsed laser sources, approaches to passively mode-locked thulium-doped lasers have been intensively investigated by making use of different kinds of saturable absorbers (SAs), such as semiconductor saturable absorber mirrors (SESAMs)[6–8], graphene or graphene oxide[9–12], and topological insulators[13,14]. With outstanding nonlinear optical properties, such as the low threshold level of saturable absorption, ultrafast recovery time, and high damage threshold, together with the advantages of easy fabrication and broad operation range[15–19], single-walled carbon nanotubes (SWCNTs) also have been applied as SAs in mode-locked lasers around 2 μm with various laser configurations in the past several years[2–4,20–22]. As SWCNTs made by conventional techniques, e.g., arc discharge, chemical vapor deposition (CVD), and laser ablation, tend to form bundled and entangled morphology the scattering is strong, thus leading to strong nonsaturable losses. To minimize the nonsaturable losses and the generated heat by the SWCNTs, researchers in Refs. [2–4,20–22] had to embed the SWCNTs in a polymer matrix. Yet the preparation of SWCNTs-polymer composites is complicated since it involves a lot of steps, for instance, selection of host polymers, dispersion of SWCNTs in solvents, and incorporation of SWCNTs into host polymers[23]. In contrast, the template method with the use of zeolite is a simpler technique to produce SWCNTs with predesigned tube structures. In addition, the SWCNTs containing zeolite are easily manipulated, which facilitates the fundamental research and practical application of SWCNTs. In our previous work, we have reported the 0.4 nm diameter and 0.3 nm diameter SWCNTs embedded in the channels of and zeolite single crystals, respectively[24–27].
In this work, we present what we believe to be the first report on a passively mode-locked thulium-doped fiber laser (TDFL) by using 0.3 nm diameter SWCNTs formed inside the channels of an MgAPO-11 (AEL) single crystal as SAs. The SWCNTs containing an AEL crystal forced a ring TDFL to produce 972 fs mode-locked pulses with a wavelength of around 1950 nm at a repetition rate of 21.05 MHz.
(AEL) is one of the crystalline microporous aluminophosphates. The framework of the crystal is constructed by alternating tetrahedral and , which form a one-dimensional (1D) elliptical channel system. The diameters of the major and minor axes are 0.65 and 0.43 nm, respectively. The adsorption of the guest molecules (to the channel wall) is relatively weak as the framework is inert chemically[27]. When crystals are used as templates to fabricate 0.3 nm diameter SWCNTs, a significant amount of organic template dipropylamine (DPA) molecules will escape from the channels during the pyrolysis process, leading to a low density and quality of 0.3 nm diameter SWCNTs in the zeolite matrix. The incorporation of Mg cations to the framework of not only increases the size of AEL single crystals, but also improves the filling density and quality of SWCNTs in the channels of AEL crystals. The detailed preparation process of MgAPO-11 single crystals can be found in Ref. [28]. The scanning electron microscope image of MgAPO-11 single crystals with a good morphology is shown in Fig. 1(a). The SWCNTs were synthesized by pyrolyzing DPA carbon precursor molecules in the channels of AEL. The carbon precursor containing AEL single crystals were heated up from room temperature to 423 K in a vacuum of for 2 h and then gradually heated up to 723 K for 4 h. Figure 1(b) shows the Raman spectra for the SWCNTs@AEL single crystal acquired using a 514 nm laser excitation. Among the low frequency modes () in the Raman spectrum, there exists a characteristic radial-breathing-mode (RBM) at that can be assigned to the (2, 2) nanotubes. The sharp RBM mode indicates that the filling density of 0.3 nm-diameter SWCNTs in the MgAPO-11 matrix is higher than that in the channels of [27]. In the high frequency range, there is a peak at that can be assigned to the tangential vibrations of carbon nanotubes.
Sign up for Chinese Optics Letters TOC. Get the latest issue of Chinese Optics Letters delivered right to you!Sign up now
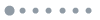
Figure 1.(a) Scanning electron microscopy image of AEL single crystals. (b) Raman spectrum of the SWCNTs@AEL single crystal.
We measured the absorption spectrum of the SWCNTs@MgAPO-11 single crystal at room temperature with a supercontinuous spectrum as the light source. The linear absorption spectrum shown in Fig. 2(a) exhibits a broadband absorption between 1800 and 2400 nm. According to the Kataura plot, the gap energy increases exponentially with the decrease of the diameter of SWCNTs[29]. The 1D density of electronic states (DOS) reveals that the electronic transition energy of (2, 2) carbon nanotubes is between [30]. Thus, we conclude that the broadband absorption shown in Fig. 2(a) may originate from the transition of vibronic levels and Grotational levels of the 0.3 nm diameter SWCNTs.
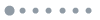
Figure 2.(a) Linear absorption spectrum of the SWCNTs@AEL single crystal. (b) Nonlinear transmittance as a function of pump peak intensity of the SWCNTs@AEL single crystal.
We also measured the power-dependent transmittance of the SWCNTs@AEL single crystal using an amplified mode-locked laser operated at a central wavelength of 1945 nm with a 21.75 MHz repetition rate and 2 ps temporal duration as the probe. Figure 2(b) gives the measurement result of the nonlinear transmission in which the fitting reveals a modulation depth of 6.9% together with a nonsaturable loss of 29.6%. The saturation intensity is estimated to be .
A passively mode-locked fiber laser around 2 μm was built with an SWCNTs@AEL crystal inserted between two fiber connectors for SA, as illustrated in Fig. 3. The inset shows the optical image of an SWCNTs@AEL crystal placed on a fiber ferrule. The laser consists of approximately 3 m of thulium-doped fiber (TDF) that was pumped by a laser diode operating at 1550 nm via a 1550/2000 wavelength division multiplexer (WDM) fiber coupler. The single-mode TDF has a core/cladding diameter of 9/125 μm with a core numerical aperture of 0.15 and peak absorption coefficient of at 1550 nm. A polarization insensitive optical isolator (ISO) was used to prevent back reflection in the cavity and ensure unidirectional laser operation. A polarization controller (PC) was included in the cavity to minimize polarization-sensitive loss in fiber couplers. Detuning the intracavity PC helps to achieve a stable passive mode-locked operation. The laser output from the oscillator is coupled out through the 10% port of a 10/90 optical coupler.
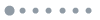
Figure 3.Schematic of the mode-locked TDFL based on 0.3 nm diameter SWCNTs.
The temporal characteristics of the laser output were monitored using a combination of a photodetector (bandwidth 10 GHz) and a 4 GHz real-time oscilloscope (Tektronix DPO70404B). The optical spectrum was measured using an optical spectrum analyzer with an operating bandwidth of 1200–2400 nm and resolution of 0.05 nm (Yokogawa AQ6375), and the radio-frequency (RF) spectrum was measured using a 3 GHz RF spectrum analyzer (Agilent, N9320A). A commercial autocorrelator (APE, PulseCheck USB) was used to characterize the mode-locked pulse’s duration.
Mode-locked operation was achieved through the adjustment of the PC at a pump power of 198 mW. Figure 4(a) shows the typical optical spectra of the mode-locked laser emissions, which centered at 1950 nm with a 3 dB bandwidth of 4.2 nm. The sharp pairs of Kelly sidebands, which are located symmetrically with respect to the central wavelength in the output spectrum, are clearly indicative of the solitary operation of the laser. It is believed that periodic spectral interference between the soliton wave and copropagating dispersive wave leads to the strong Kelly sidelobes[31].
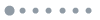
Figure 4.(a) Optical spectrum of the mode-locked TDFL based on 0.3 nm diameter SWCNTs at the pump power of 251 mW. (b) Autocorrelation trace of the output pulse with FWHM of 972 fs. (c) RF spectrum and the output pulse trains (inset). (d) CW laser output spectrum of the TDFL without the SWCNTs@AEL single crystal in the cavity.
Figure 4(b) shows the autocorrelation trace for the proposed mode-locked TDFL. The pulse has a full width at half-maximum (FWHM) width of about 972 fs, corresponding to the time-bandwidth product of , which is quite close to the transform-limited value of 0.315 for a soliton pulse. At the pump power of 420 mW, the measured average output power is 2.3 mW, which corresponds to the pulse energy and peak power of 109 pJ and 112 W, respectively.
Figure 4(c) presents a measurement made within a scanning range of 7 MHz and a resolution bandwidth of 1 kHz. The fundamental peak located at the cavity repetition rate of 21.05 MHz (period ) has a peak-to-background ratio of 61 dB. It is indicative of stable mode-locked operation and low jittering of the pulse trains. The output pulse trains of the laser emission, which were measured by a digital oscilloscope, are shown in the inset of Fig. 4(c). The mode-locking operation was stable while the pump power was in the range of 198–420 mW. Long-term stability measurement was not made; nevertheless, for several hours during experiments the laser operation is stable. Once the applied pump power was higher than 420 mW, pulse splitting was observed, which is in agreement with soliton-type pulse behavior[32,33].
We have also verified whether the soliton mode-locked operation of the TDFL was dependent on the 0.3 nm diameter SWCNTs. In the case of absence of the SWCNTs@AEL absorber in the cavity, the laser always works in a continuous wave (CW) regime with a wavelength at 1952 nm [Fig. 4(d)], although the PC was rotated and the pump power was adjusted on a large scale. The result confirmed that the mode-locked state was caused by the 0.3 nm diameter SWCNTs embedded in MgAPO-11 matrix.
In conclusion, an all-fiber mode-locked TDFL ring oscillator with the help of 0.3 nm diameter SWCNTs formed inside the MgAPO-11 matrix is demonstrated. The laser delivers soliton pulses centering at 1950 nm with a spectral width of 4.2 nm. Single pulses with an FWHM width of 972 fs and 21.05 MHz mode-locked pulse train were also observed. Our results indicate that 0.3 nm diameter SWCNTs SAs synthesized by the template method with the use of zeolite are well suited for passive mode-locking fiber lasers around 2 μm.
References
[1] J. F. Wu, Z. D. Yao, J. Zong, S. B. Jiang. Opt. Lett., 32, 638(2007).
[2] M. A. Solodyankin, E. D. Obraztsova, A. S. Lobach, A. I. Chernov, A. V. Tausenev, V. I. Konov, E. M. Dianov. Opt. Lett., 33, 1336(2008).
[3] K. Kieu, F. W. Wise. IEEE Photonics Technol. Lett., 21, 128(2009).
[4] A. Schmidt, P. Koopmann, G. Huber, P. Fuhrberg, S. Y. Choi, D. Yeom, F. Rotermund, V. Petrov, U. Griebner. Opt. Express, 20, 5313(2012).
[5] S. M. Azooz, F. Ahmad, H. Ahmad, S. W. Harun, B. A. Hamida, S. Khan, A. Halder, M. C. Paul, M. Pal, S. K. Bhadra. Chin. Opt. Lett., 13, 030602(2015).
[6] F. Fusari, A. A. Lagatsky, G. Jose, S. Calvez, A. Jha, M. D. Dawson, J. A. Gupta, W. Sibbett, C. T. A. Brown. Opt. Express, 18, 22090(2010).
[7] L. M. Yang, P. Wan, V. Protopopov, J. Liu. Opt. Express, 20, 5683(2012).
[8] H. H. Li, J. Liu, Z. C. Cheng, J. Xu, F. Z. Tan, P. Wang. Opt. Express, 23, 6292(2015).
[9] J. Ma, G. Q. Xie, P. Lv, W. L. Gao, P. Yuan, L. J. Qian, H. H. Yu, H. J. Zhang, J. Y. Wang, D. Y. Tang. Opt. Lett., 37, 2085(2012).
[10] Q. Q. Wang, T. Chen, B. Zhang, M. S. Li, Y. F. Lu, K. P. Chen. Appl. Phys. Lett., 102, 131117(2013).
[11] M. W. Jung, J. H. Koo, P. Debnath, Y. W. Song, J. H. Lee. Appl. Phys. Express, 5, 112702(2012).
[12] J. Boguslawski, J. Sotor, G. Sobon, R. Kozinski, K. Librant, M. Aksienionek, L. Lipinska, K. M. Abramski. Photon. Res., 3, 119(2015).
[13] M. W. Jung, J. S. Lee, J. H. Koo, J. H. Park, Y. W. Song, K. Lee, S. B. Lee, J. H. Lee. Opt. Express, 22, 7865(2014).
[14] X. Zou, Y. Leng, Y. Li, Y. Feng, P. Zhang, Y. Hang, J. Wang. Chin. Opt. Lett., 13, 081405(2015).
[15] P. Avouris, M. Freitag, V. Perebeinos. Nat. Photonics, 2, 341(2008).
[16] S. Tatsuura, M. Furuki, Y. Sato, I. Iwasa, M. Tian, H. Mitsu. Adv. Mater., 15, 534(2003).
[17] S. Y. Set, H. Yaguchi, Y. Tanaka, M. Jablonski. J. Lightwave Technol., 22, 51(2004).
[18] Y. C. Chen, N. R. Raravikar, Y. P. Zhao, L. S. Schadler, P. M. Ajayan, T. M. Lu, G. C. Wang, X. C. Zhang. Appl. Phys. Lett., 81, 975(2002).
[19] W. B. Cho, J. H. Yim, S. Y. Choi, S. Lee, A. Schmidt, G. Steinmeyer, U. Griebner, V. Petrov, D.-I. Yeom, K. Kim, F. Rotermund. Adv. Funct. Mater., 20, 1937(2010).
[20] W. B. Cho, A. Schmidt, J. H. Yim, S. Y. Choi, S. Lee, F. Rotermund, U. Griebner, G. Steinmeyer, V. Petrov, X. Mateos, M. C. Pujol, J. J. Carvajal, M. Aguiló, F. Díaz. Opt. Express, 17, 11007(2009).
[21] S. W. Harun, N. Saidin, D. I. M. Zen, N. M. Ali, H. Ahmad, F. Ahmad, K. Dimyati. Chin. Phys. Lett., 30, 094204(2013).
[22] M. Zhang, E. J. R. Kelleher, T. H. Runcorn, V. M. Mashinsky, O. I. Medvedkov, E. M. Dianov, D. Popa, S. Milana, T. Hasan, Z. Sun, F. Bonaccorso, Z. Jiang, E. Flahaut, B. H. Chapman, A. C. Ferrari, S. V. Popov, J. R. Taylor. Opt. Express, 21, 23261(2013).
[23] T. Hasan, Z. P. Sun, F. Q. Wang, F. Bonaccorso, P. H. Tan, A. G. Rozhin, A. C. Ferrari. Adv. Mater., 21, 3874(2009).
[24] N. Wang, Z. K. Tang, G. D. Li, J. S. Chen. Nature, 408, 426(2000).
[25] Z. K. Tang, L. Y. Zhang, N. Wang, X. X. Zhang, G. H. Wen, G. D. Li, J. N. Wang, C. T. Chan, P. Sheng. Science, 292, 2462(2001).
[26] Z. K. Tang, J. P. Zhai, Y. Y. Tong, X. J. Hu, R. Saito, Y. J. Feng, P. Sheng. Phys. Rev. Lett., 101, 047402(2008).
[27] J. P. Zhai, I. L. Li, S. C. Ruan, Z. K. Tang. Microporous Mesoporous Mater., 124, 15(2009).
[28] Y. P. Chen, J. P. Zhai, X. T. Xu, I. L. Li, S. C. Ruan, Z. K. Tang. CrystEngComm., 16, 2984(2014).
[29] H. Kataura, Y. Kumazawa, Y. Maniwa, I. Umezub, S. Suzuki, Y. Ohtsuka, Y. Achiba. Synth. Met., 103, 2555(1999).
[30] S. Maruyama. For one-dimensional DOS for each chiralities(2002).
[31] S. M. J. Kelly. Electron. Lett., 28, 806(1992).
[32] K. Kieu, M. Mansuripur. Opt. Lett., 32, 2242(2007).
[33] H. Jeong, S. Y. Choi, E. Jeong, S. J. Cha, F. Rotermund, D. Yeom. Appl. Phys. Express, 6, 052705(2013).