Author Affiliations
1School of Information Science and Engineering, and Shandong Provincial Key Laboratory of Laser Technology and Application, Shandong University, Qingdao 266237, China2Key Laboratory of Micro-Nano Measurement-Manipulation and Physics (Ministry of Education), Department of Applied Physics, Beihang University, Beijing 100191, China3Shenzhen Research Institute of Shandong University, Shenzhen 518057, China4State Key Laboratory of Crystal Materials, Institute of Crystal Materials, Shandong University, Jinan 250100, China5Key Laboratory of Transparent and Opto-Functional Inorganic Materials, Artificial Crystal Research Center, Shanghai Institute of Ceramics, Chinese Academy of Sciences, Shanghai 201899, China6School of Physics Science and Engineering, Institute for Advanced Study, Tongji University, Shanghai 200092, Chinashow less
Abstract
A dual-wavelength synchronously mode-locked homogeneously broadened bulk laser operating at 1985.6 and 1989 nm is presented for the first time, to the best of our knowledge, which delivers a maximum output power of 166 mW and a repetition rate of 85 MHz. The pulse duration was measured to be 16.8 ps by assuming a pulse shape. The recorded autocorrelation trace showed frequency beating signals with an interval of 3.8 ps and a full width at half-maximum duration of 2 ps, corresponding to an ultrahigh beating frequency of about 0.26 THz, which agrees well with the frequency difference of the emitted two spectral peaks. The results indicated that such a kind of dual-wavelength mode-locked Tm:YAlO3 laser could be potentially used for generating terahertz radiations.Dual-wavelength ultrafast lasers are gaining more and more attention, owing to their exceptional properties of synchronous emission of different-color beating signals with ultrahigh beating frequency up to terahertz (THz) caused by optical beating. Such lasers are highly required for applications needing very fast acquisition time such as fluid dynamic measurements[1]. Additionally, pulsed lasers with dual-wavelength output also play a vital role in generating THz waves, which have become a hot topic due to their unique applications including interstellar and intragalactic observation, T-ray imaging, and broad-band secure communications[2,3]. Employing -doped sapphire and -doped crystals as gain media, dual-wavelength synchronously mode-locked lasers operating at and have been widely demonstrated. Beating pulses with several hundred femtosecond pulse duration and THz-level ultrahigh beating frequency have been experimentally generated[4–12]. Compared with the near-infrared laser emissions, lasers are more striking due to their characteristics of eye safety and special wavelength, which makes it gain numerous free space applications including coherent laser radar, gas sensing, medical surgery, and pumping of optical parametric oscillators (OPOs)[13,14]. However, very few reports on dual-wavelength ultrafast pulsed lasers at have been found. In 2015 and 2017, ultrafast beating pulses with THz-level beating frequency were delivered from dual-wavelength mode-locked (Tm:CYA) and Tm, lasers, respectively[15,16]. Tm:CYA and Tm, were disordered crystals, in which the fluorescence spectra were inhomogeneously broadened and therefore beneficial for synchronous dual-wavelength operation. However, the disordered structure has detrimental impact on the thermal property of the crystals, as described in Ref. [17]; thus, mass disorder would increase the thermal resistivity. For example, in our previous work based on Tm,, a low damage threshold of several kilowatts per centimeter (kW/cm2) was observed[18], which meant the broad emission spectra were at the cost of decreasing the thermal conductivity. So much more efforts should still be paid on exploring novel dual-wavelength ultrafast laser systems, especially based on the crystals with excellent mechanical and thermal properties.
A Tm-doped (Tm:YAP) crystal, a widely investigated gain material, has many favorable features including structural anisotropy, long Y-O bonds, and natural birefringence, which makes it free of the harmful thermally induced depolarization phenomenon[19–21]. Compared with the (Tm:YAG) crystal, the Tm:YAP crystal not only has similar mechanical properties, but twice the emission cross section[21]. In recent years, continuous wave (CW), -switching, and single-wavelength mode-locking (ML) lasing operations of the Tm:YAP crystals have been experimentally demonstrated[21–27]. As can be seen from the fluorescence spectrum of the Tm:YAP crystal in Ref. [28], there are multiple emission peaks located in the spectral region originating from its anisotropic structure, which benefits the generation of dual- or multi-wavelength emissions. In 2016, dual-wavelength operation was experimentally realized in a black phosphorus passively -switched Tm:YAP laser[24], well verifying the ability of the Tm:YAP crystal in generating dual-wavelength laser radiations. Considering the advantages of the Tm:YAP crystal compared with the disordered crystals mentioned above, a synchronous dual-wavelength ultrafast laser using the Tm:YAP crystal is also expected. However, there are no reports on the dual-wavelength ultrafast laser operations based on the Tm:YAP crystal, to the best of our knowledge.
In this Letter, a diode-pumped dual-wavelength synchronously mode-locked Tm:YAP laser at 1985.6 and 1989 nm was experimentally demonstrated. The autocorrelation trace was recorded, and the beating signals were observed, which were found to have an ultrahigh beating frequency of 0.26 THz and full width at half-maximum (FWHM) duration of 2 ps. The results indicated a potential way to obtain efficient THz radiation by further using a suitable difference frequency generation (DFG) method.
Sign up for Chinese Optics Letters TOC. Get the latest issue of Chinese Optics Letters delivered right to you!Sign up now
The schematic setup for the diode-pumped mode-locked Tm:YAP laser was depicted in Fig. 1. A laser diode emitting at 793 nm was employed as a pump source and fiber coupled with a core diameter of and a numerical aperture of 0.22. Through a 1:1 imaging module, the pump light was focused into the Tm:YAP crystal. A 1 at. %-doped -cut Tm:YAP crystal was enclosed with indium foil and mounted in a water-cooled copper heat sink with constant temperature of 8°C to efficiently remove the accumulated heat inside the crystal induced by the quantum defect and imperfect absorbance of pump light. M1 was an input flat mirror anti-reflection (AR) coated at the pump wavelength and high-reflection (HR) coated at the laser wavelength. The crystal was placed as close as possible to M1, while maintaining good mode-matching condition between the pump light and laser beam. This intentional configuration design was to enhance the spatial hole burning effect inside the Tm:YAP crystal, which would be helpful for forming the population grating, thus inhomogeneously broadening the gain bandwidth[29] and generating short laser pulses. M2 and M5 were concave mirrors with curvature radii of 500 and 200 mm, respectively. M3 and M4 were flat Gires–Tournois interferometer (GTI) mirrors with group delay dispersion (GDD) of at for dispersion compensation. A flat mirror with low transmission rate of 1% at was employed as the output coupler. Due to the dual-channel setup, the total output coupling efficiency was 2%. The semiconductor saturable absorber mirror (SESAM) was placed at the end of the cavity to take advantage of the second beam waist formed by mirror M5. The laser spot radii on the Tm:YAP crystal and SESAM were calculated to be and in tangential and sagittal planes, respectively. The SESAM (Batop Inc, Germany) had a modulation depth of 1%, a recovery time of 10 ps, a saturation fluence of , and a non-saturable loss of 1.2%.
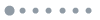
Figure 1.Experimental setup of the dual-wavelength mode-locked Tm:YAP laser.
Firstly, with an HR flat mirror substituting SESAM, the CW characteristics of the Tm:YAP laser were investigated. The threshold absorbed pump power was measured to be 1.1 W. The average output powers increased almost linearly with the absorbed pump powers, as shown in Fig. 2, corresponding to a slope efficiency of 21.7%. However, at high pump level (beyond 4.5 W of absorbed pump power), the average output powers somehow showed saturation tendency. In comparison with that, no obvious saturation tendency was observed in the later CWML operation. Considering the same pump level for CW and CWML operations, the saturation tendency only happening in the CW regime was not mainly attributed to the excess heat generated inside the Tm:YAP crystal, but the gain saturation effect induced by the faster decrease of the ground state population intensity in the CW regime than that in the CWML case, which was not only due to the low ions doping concentration of 1 at. % but the low intracavity loss of CW operation under high pump levels. Substituting the HR end mirror with SESAM, the threshold absorbed pump power increased to 2.66 W, and the laser ran into the -switched ML (QML) regime directly. Under the absorbed pump power of 5 W, a total maximum output power of 166 mW from the two output directions was obtained. To prevent the SESAM from being damaged, the pump power was not enhanced further, which subsequently limited the maximum output power.
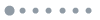
Figure 2.Average output power versus absorbed pump power of the Tm:YAP laser in CW and CWML regimes.
With a fast InGaAs photodetector (EOT, ET-5000, USA) and digital oscilloscope (1 GHz bandwidth, Tektronix DPO 7102, USA), temporal pulse trains from the mode-locked Tm:YAP laser were monitored and shown in Fig. 3. The pulse stability can be confirmed by the smooth profile in scale of and the negligible time jitter of the discrete laser pulses in the scale of 20 ns/div.
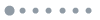
Figure 3.Pulse trains from the mode-locked Tm:YAP laser.
Figure 4 shows the first beat note of the radio frequency (RF) spectrum at 85 MHz with a resolution bandwidth of 50 kHz. The single beat note with an extinction ratio of 52 dBc confirmed the clear CWML operation without -switching instability. In addition, no spurious modulation was found in the 1 GHz wide-span RF measurement, which indicated the single-pulse operation of the mode-locked laser, as shown in the inset of Fig. 4. However, the fast decline of the beat note amplitudes was observed, to which we attributed two aspects: one is the loss of the employed RG-58-type coaxial cable for connecting the photodetector and RF analyzer, since the larger loss for higher frequencies in such types of cables would reduce the bandwidth, and the other one may come from the saturation effect in the photodetector, which would cause the bandwidth reduction of the photodetector. The single-pulse operation that could be confirmed either by the oscillagram (Fig. 3) or RF spectrum (Fig. 4) strongly evidenced the temporal synchronization of the two sets of mode-locked pulse trains with different colors.
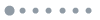
Figure 4.RF spectra for the mode-locked Tm:YAP laser around the fundamental beat note of 85 MHz with bandwidth of 50 kHz and (inset) in a wide span of 1 GHz with a resolution bandwidth of 5 MHz.
Using a laser spectrometer (APE WaveScan, APE Inc.) with a resolution of 0.4 nm and a second-harmonic generation (SHG)-based autocorrelator (APE GmbH, Pulse Check 150), the output spectrum and autocorrelation trace have been measured and shown in Fig. 5. By assuming a pulse shape, the pulse duration was measured to be about 16.8 ps. Moreover, the optical beating pattern of the two-color laser pulses could be clearly observed from the autocorrelation trace. The interval period T between the beating signals was found to be 3.8 ps, which is consistent with the theoretical value of 3.87 ps determined by , considering the frequency difference of 0.258 THz between the two output spectral peaks of 1985.6 and 1989 nm. In addition, the FWHM duration of the beating signal was found to be 2 ps. The GTI mirrors would be crucial for the realization of synchronous dual-wavelength ML, which requires the balances of dispersion and nonlinearity, gain and loss, as well as saturable absorption of the SESAM[30].
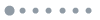
Figure 5.Autocorrelation trace for the dual-wavelength synchronously mode-locked Tm:YAP laser. Inset: output spectrum.
In conclusion, a dual-wavelength synchronously mode-locked Tm:YAP laser operating at 1985.6 and 1989 nm has been experimentally demonstrated with a maximum output power of 166 mW and a pulse repetition rate of 85 MHz. The pulse duration was measured to be 16.8 ps by assuming a pulse shape. Frequency beating was observed from the autocorrelation trace, and the beating signals showed an interval of 3.8 ps and an FWHM duration of 2 ps, corresponding to an ultrahigh beating frequency of about 0.26 THz, which agrees well with the frequency difference of the emitted two spectral peaks. To the best of our knowledge, this is the first experimental demonstration of a dual-wavelength mode-locked laser based on a kind of homogeneously broadened gain medium, which has paved a novel potential way of generating THz radiation by a suitable DFG method.
References
[1] B. S. Thurow, N. Jiang, W. R. Lempert. Meas. Sci. Technol., 24, 012002(2012).
[2] P. H. Siegel. IEEE Trans. Microwave, Theory Tech., 50, 910(2002).
[3] K. Kang, L. Zhang, T. Wu, K. Li, C. Zhang. Chin. Opt. Lett., 16, 110401(2018).
[4] G. Q. Xie, D. Y. Tang, H. Luo, H. J. Zhang, H. H. Yu, J. Y. Wang, X. T. Tao, M. H. Jiang, L. J. Qian. Opt. Lett., 33, 1872(2008).
[5] A. Agnesi, F. Pirzio, G. Reali, A. Arcangeli, M. Tonelli, Z. Jia, X. Tao. Appl. Phys. B, 99, 135(2010).
[6] J.-L Xue, B.-Y. Zhang, Y. Yang, H. Yang, S.-D. Liu. Appl. Phys. B, 107, 53(2012).
[7] F. Zhang, X. Fan, J. Liu, F. Ma, D. Jiang, S. Pang, L. Su, J. Xu. Opt. Mater. Express, 6, 1513(2016).
[8] C. Feng, H. Zhang, Q. Wang, J. Fang. Laser Phys. Lett., 14, 045804(2017).
[9] X. Sun, J. He, Z. Jia, J. Ning, R. Zhao, X. Su, Y. Wang, B. Zhang, K. Yang, S. Zhao. RSC Adv., 7, 32044(2017).
[10] W. H. Knox, F. A. Beisser. Opt. Lett., 17, 1012(1992).
[11] Z. Zhang, T. Yagi. Opt. Lett., 18, 2126(1993).
[12] C. J. Zhu, J. F. He, S. C. Wang. Opt. Lett., 30, 561(2005).
[13] E. Ji, M. Nie, Q. Liu. Chin. Opt. Lett., 15, 091402(2017).
[14] B. Ma, K. Kafka, E. Chowdhuiy. Chin. Opt. Lett., 15, 051901(2017).
[15] L. C. Kong, Z. P. Qin, G. Q. Xie, X. D. Xu, J. Xu, P. Yuan, L. J. Qian. Opt. Lett., 40, 356(2015).
[16] L. Kong, Z. Qiao, G. Xie, Z. Qin, B. Zhao, H. Yu, L. Su, J. Ma, P. Yuan, L. Qian. Opt. Express, 25, 21267(2017).
[17] J. Garg, N. Bonini, B. Kozinsky, N. Marzari. Phys. Rev. Lett., 106, 045901(2011).
[18] X. Liu, K. Yang, S. Zhao, T. Li, C. Luan, X. Guo, B. Zhao, L. Zheng, L. Su, J. Xu, J. Bian. Opt. Lett., 42, 2567(2017).
[19] G. A. Massey. Appl. Phys. Lett., 17, 213(1970).
[20] Y. Lu, J. Wang, Y. Yang, Y. Dai, A. Dong, B. Sun. J. Alloys Compd., 429, 296(2007).
[21] I. F. Elder, J. Payne. Appl. Opt., 36, 8606(1997).
[22] J. Liu, Y. G. Wang, Z. S. Qu, L. H. Zheng, L. B. Su, J. Xu. Laser Phys. Lett., 9, 15(2012).
[23] J. Hou, B. Zhang, X. Su, R. Zhao, Z. Wang, F. Lou, J. He. Opt. Commun., 347, 88(2015).
[24] H. Zhang, J. He, Z. Wang, J. Hou, B. Zhang, R. Zhao, K. Han, K. Yang, H. Nie, X. Sun. Opt. Mater. Express, 6, 2328(2016).
[25] H. Wan, W. Cai, F. Wang, S. Jiang, S. Xu, J. Liu. Opt. Quantum Electron., 48, 11(2016).
[26] B. Cole, L. Goldberg, A. D. Hays. Opt. Lett., 43, 170(2018).
[27] S. Zhang, X. Zhang, J. Huang, T. Wang, J. Dai, G. Dong. Laser Phys., 28, 035804(2018).
[28] Y. Lu, Y. Dai, Y. Yang, J. Wang, A. Dong, B Sun. J. Alloys Compd., 453, 482(2008).
[29] C. L. Tang, H. Statz, G. deMars. J. Appl. Phys., 34, 2289(1963).
[30] W. Q. Zhang, D. G. Lancaster, T. M. Monro, S. A. Vahid. Sci. Rep., 8, 7821(2018).