
- Photonics Research
- Vol. 11, Issue 2, 159 (2023)
Abstract
1. INTRODUCTION
The random fiber laser (RFL), which was proposed by Turitsyn
Various kinds of RFL schemes have been demonstrated to realize wavelength tunability [19,20,24–28], including inserting a tunable filter into the cavity [20], employing an optical grating filter [26], and combining a fiber Fabry–Perot cavity with a Mach–Zehnder interferometer [27]. A tunable pump source is combined with the cascaded stimulated Raman scattering process to further widen the wavelength tuning range [25]. A cascaded random Raman fiber laser using a comb filter can also realize spectral manipulation [28]. Apart from the selection of wavelength, there are already a variety of structures for implementing multi-wavelength generation of fiber lasers. Common methods include the stimulated Brillouin scattering effect [29,30], four-wave mixing effect [31], Mach–Zehnder interferometer [32], Sagnac loop mirror [33], Fabry–Perot interferometer [34], fiber Bragg grating [35,36], two-dimensional materials [37], ultra-long-period grating [38], nonlinear amplifying loop mirror [39], and Loyt filter [22]. However, flexible wavelength tuning and multi-wavelength generation can hardly be obtained simultaneously.
Fortunately, the acousto-optic tunable filter (AOTF) can offer effective access to generate multi-wavelength lasing while achieving flexible wavelength choices and wavelength-dependent loss. The central wavelength and corresponding loss can be controlled by designing radio frequency (RF) signals [40]. Since its advent, AOTF has been widely applied in different fields [40–43]. Flexible multi-wavelength tuning has been actualized based on an ytterbium-doped fiber, whose tuning range reaches 1032–1111 nm [44,45]. In addition, a tunable multi-wavelength erbium-doped fiber laser has also been realized with a tuning range from 1524 to 1567 nm [46]. Overall, few wavelength-tunable fiber lasers have been realized based on this multifunction device [47,48]. Furthermore, there is no report of an AOTF used for cavity-free RFL to achieve flexible multi-wavelength output. But it is anticipated that the flexible tuning capability makes AOTF a versatile device for spectral filtering and manipulation, which enables a multi-wavelength RFL employed by an AOTF to achieve high-power-spectrum editable random laser output.
Sign up for Photonics Research TOC. Get the latest issue of Photonics Research delivered right to you!Sign up now
In this paper, a spectrum-customizable multi-wavelength RFL enabled by an AOTF is demonstrated. The central wavelength can be tuned from 1114.5 to 1132.5 nm by adjusting the frequency of the RF signal loaded on the AOTF. In addition, the number of lasing wavelengths, which is controlled by the channel number of RF signals, can be adjusted from one to five. The linewidth can be widened to 4.5 nm, and each wavelength component can be tuned independently to achieve spectral-flexible output.
2. EXPERIMENTAL SETUP
The schematic of the spectral-flexible multi-wavelength RFL is shown in Fig. 1. A homemade high-power amplified spontaneous emission (ASE) source is adopted as the pump light, whose working bandwidth is 15.2 nm and centered at 1065 nm [49]. A roll of 3 km passive fiber is applied to provide Raman gain and random distributed feedback. The core and cladding diameters of the passive fiber are 9 and 125 μm, respectively. The numerical aperture (NA) of the fiber core is 0.14, and the attenuation coefficient is about 0.7 dB/km when the central wavelength is between 1050 and 1150 nm. A 1070/1120 nm wavelength division multiplexer (WDM) is inserted between the pump source and passive fiber to separate the backward scattering Stokes light component from the pump light. The 1070 nm port of WDM is connected with the ASE source while the 1120 nm port is connected with a feedback loop composed of a circulator and an AOTF. The backward Stokes light component is used as feedback light that enters the circulator from port 2 and is output at port 3, and then it is filtered by the AOTF. After filtering, the feedback light enters the circulator from port 1 and is output at port 2, and then is injected into the passive fiber through the WDM. An optical spectrum analyzer (OSA) and a power monitor (PM) are respectively adopted to measure the output spectrum and power after the output port.
Figure 1.Schematic diagram and operation principle of multi-wavelength RFL.
Due to the adoption of a wide bandwidth pump source, the gain spectrum of the passive fiber is flat and has a characteristic of high-middle and low-edge gain [22]. In the operation, to obtain multi-wavelength outputs with equal amplitudes, we adjust the applied voltage to make the loss at the middle wavelengths higher and the loss at the edge wavelengths lower in the AOTF transmission spectrum. The spectra in Fig. 1 show the process of multi-wavelength output with equal amplitudes enabled by controlling the transmission spectrum of AOTF. The edges of the filtered spectrum have higher transmittance, which corresponds to the wave band of the gain spectrum having lower gain. Conversely, the filtering loss is higher in the region with higher gain. The interaction between the two results in multi-wavelength output with equal amplitude.
3. RESULTS AND DISCUSSION
A. Single-Wavelength Output and Central Wavelength Tuning
First, we measured the transmission spectrum of the AOTF. The first-order Raman laser that corresponds to the common 1070 nm pump source is about 1123 nm in this investigation, and we display the corresponding filtered spectrum of AOTF in Fig. 2(a). The full width at half maximum (FWHM) linewidth of the main peak is 2.0 nm, and several sidebands appear beside the main peak. The wavelength interval between the main peak and secondary peak is about 5 nm, and the amplitude difference is about 12 dB.
Figure 2.(a) Filtered spectrum of AOTF at the central wavelength of 1123.0 nm. (b) Power evolution under different pump powers at the central wavelength of 1123 nm. Output spectrum of 1123 nm lasing at pump power levels of (c) 8.45 W and (d) 8.84 W.
Then, we measured the power and spectral evolution characteristics when the pump wavelength is about 1070 nm and the signal light wavelength is 1123 nm. As shown in Figs. 2(b) and 2(c), it can be observed that when the pump power exceeds 3.87 W, the 1070 nm component of the output power decreases rapidly and transforms into a 1.1 μm random laser quickly. When the pump power reaches 8.45 W, the 1.1 μm random laser achieves a maximal power of 4.67 W, corresponding to a conversion efficiency of 55.3%. As the passive fiber can introduce about 40% power loss with a transmission loss coefficient of about 0.7 dB/km and 3 km length, the conversion efficiency is mainly limited by the loss of the passive fiber. Figure 2(c) depicts the spectrum when the 1.1 μm random laser achieves its maximum (4.67 W). The central wavelength is 1123 nm and the FWHM is 1.08 nm. It is obvious that the sidebands of the feedback light are suppressed and the main peak is narrowed for the gain competition in the passive fiber [50]. The SNR is more than 30 dB, and the measured highest spectral purity is close to 100% in this case, where the ASE pump source contributes significantly. With further growth of pump power, the 1.1 μm random laser component will convert into the next order, and the output power of the first order Stokes component will be reduced, as presented in Fig. 2(d). Since the wavelength component of the next order is beyond the operating range of devices such as circulators, the pumping power was not further increased to avoid system damage. This means that the output power is limited by the generation of the next order Stokes component. By shortening the length of the passive fiber and increasing the core diameter, the stimulated Raman scattering threshold can be effectively increased, resulting in higher output power. However, it also greatly increases the power of the backward scattering light, which may exceed the power tolerance of AOTF. Hence, further optimization of the system structure is needed to achieve higher output power.
In this scheme, central wavelength tuning is easily achieved just by adjusting the frequency of the RF signal. As the frequency of the RF signal is continuously adjusted from 60.766 to 59.69 MHz, the central wavelength of the RFL varies from 1115 to 1132 nm, accordingly. Figure 3(a) displays the output spectra at different central wavelengths; the FWHM linewidth of each spectrum is between 1.08 and 1.32 nm. The maximal output power is also changed with the adjustment of the central wavelength. The power fluctuations are less than 18% over the entire tuning range. With the improvement of output power at the margin of the tuning range, a significant spontaneous Raman peak occurs. As revealed in Fig. 3(b), the component of spontaneous Raman lasing appears at the wavelength corresponding to the gain peak of pump light. The SNR reduces rapidly to 13 and 10 dB, respectively. Therefore, the wavelength tuning range is limited by the reduced SNR due to spontaneous Raman radiation.
Figure 3.(a) Output spectra at different central wavelengths and corresponding maximum output power. (b) Spontaneous Raman lasing appears while the central wavelengths are 1114.4 and 1132.5 nm.
B. Dual-Wavelength Output Characteristics and Linewidth Adjustment
On the basis of an 18 nm tuning range with the introduced RF signal, dual-wavelength output can easily be achieved by adding a second channel; the corresponding central wavelength is decided by the frequencies of the dual-channel RF signal. Figure 4(a) displays dual-wavelength output spectra with a wavelength interval of 10 nm centered at 1118 and 1128 nm, whose SNR is more than 25 dB, and the major frequencies are located at 60.585 and 59.98 MHz. When the major frequencies are tuned to 60.357 and 60.06 MHz, the SNR decreases to about 15 dB and the interval is adjusted to 4 nm. The spectrum is displayed in Fig. 4(b). The output power is about 4.73 W with a fluctuation ratio of 4.53% when operating wavelength is tuned. Actually, we can obtain dual-wavelength outputs in any combination within the tuning range by adjusting the RF signal frequencies of both channels. It should be noted that the SNR will be reduced as the wavelength interval is further decreased. However, the corresponding lasing tends to merge so as not to be distinguished when the signal frequency of different channels is similar.
Figure 4.Spectrum of dual-wavelength output with the interval of (a) 10 nm and (b) 4 nm.
In the investigation, we further reduced the RF signal frequency interval to control the linewidth. A dual-channel RF signal, whose frequencies are located at 60.39 and 60.22 MHz with radio amplitudes of 23 and 24, is loaded on the AOTF. The corresponding filter spectrum of AOTF is plotted in Fig. 5(a). In such a situation, the spectral components corresponding to the two frequencies overlap and merge into a broader spectrum with an FWHM linewidth of about 5.7 nm. The laser output spectrum at this parameter condition is shown in Fig. 5(b), with an FWHM linewidth of about 4 nm, which may be influenced by the narrowing of the spectrum due to gain competition. As the number of RF frequencies is further increased to five, we obtain the output spectrum, as shown in Fig. 5(c). The FWHM linewidth of the output random laser increases to 4.5 nm, which is broader with a smaller percentage increase compared to dual-channel output, which may be the result of gain competition.
Figure 5.(a) Filtered spectrum with dual-channel RF signal. Output spectra with FWHM of (b) 4 nm and (c) 4.5 nm.
C. Multi-Wavelength Output and Spectral Shape Control
Enabled by this multifunctional AOTF, multi-wavelength tuning is also achievable. As the channel number of the RF signals increases, multi-channel filtering can be realized. Figure 6(a) displays the tri-wavelength output with equal amplitudes and five-wavelength output with similar amplitudes, whose difference of relative wavelength is less than 1 dB. The tri-wavelength lasing spectrum is positioned at 1116, 1123, and 1130 nm, while the five-wavelength one is centered at 1115, 1119, 1123, 1127, and 1131 nm. The output powers are 5.17 and 5.03 W for tri-wavelength and five-wavelength output, respectively. Since the gain spectrum is not flat, we optimized the RF signal amplitude to obtain the transmission spectra, as shown in Fig. 6(b), in the experiments, to make up for the gain spectrum and get a laser line of equal amplitude.
Figure 6.(a) Spectra of tri-wavelength and five-wavelength output with equal/similar amplitude. (b) Corresponding filtered spectra.
In addition to the equal intensity multi-wavelength operation, variable intensity is also available from this multifunctional AOTF-based RFL. The output spectrum can be customized by adjusting the loading RF signals. Based on the five-wavelength equal-amplitude output, a spectrum with particular shapes, as plotted in Fig. 7, can be ordered by changing the radio amplitude. All spectra were measured at a pump power of 9.83 W, and the maximum power changes a little (
Figure 7.Five-wavelength output with customized shape.
4. CONCLUSION
In conclusion, we have demonstrated an AOTF-enabled RFL with the characterization of wavelength selection and intensity tuning at the same time. The central wavelength can be operated in the range of 1114.5–1132.5 nm, and spectral channel tuning can also be realized with a maximal number of five. Even linewidth can be widened to 4.5 nm by the designed RF signal. Optimized loss of the filter makes up for the gain spectrum, leading to equal amplitude multi-wavelength outputs. Further adjustments allow us to obtain a customized spectral envelope. This spectrum-customizable multi-wavelength RFL has the potential of broadening the tuning wave band of tunable fiber lasers and promoting multi-wavelength RFLs’ practicability in sensing, telecommunications, and precise spectroscopy.
References
[40] P. C. Shardlow, J. Ward, C. Pannell, S. Valle, W. A. Clarkson. Null-frequency-shift acousto-optic tunable filter for wavelength tuning of a tm fibre laser. European Conference on Lasers and Electro-Optics–European Quantum Electronics Conference, CJ_14_16(2015).
[42] K. V. Zaichenko, B. S. Gurevich. Spectral selection using acousto-optic tunable filters for the skin lesions diagnostics. European Conferences on Biomedical Optics (ECBO), EM1A.8(2021).
[43] A. Machikhin. Acousto-optical tunable filters: applications in 3D imaging and multi-wavelength digital holography. OSA Imaging and Applied Optics Congress (3D, COSI, DH, ISA, pcAOP), DM5E.1(2021).
[46] N. Hashimoto, H. Miyata, Y. Takasu, G. Han, T. Uematsu, T. Nakazawa. Tunable erbium-doped fiber ring laser using acousto-optic tunable filter. Integrated Photonics Research, ITuG4(2001).
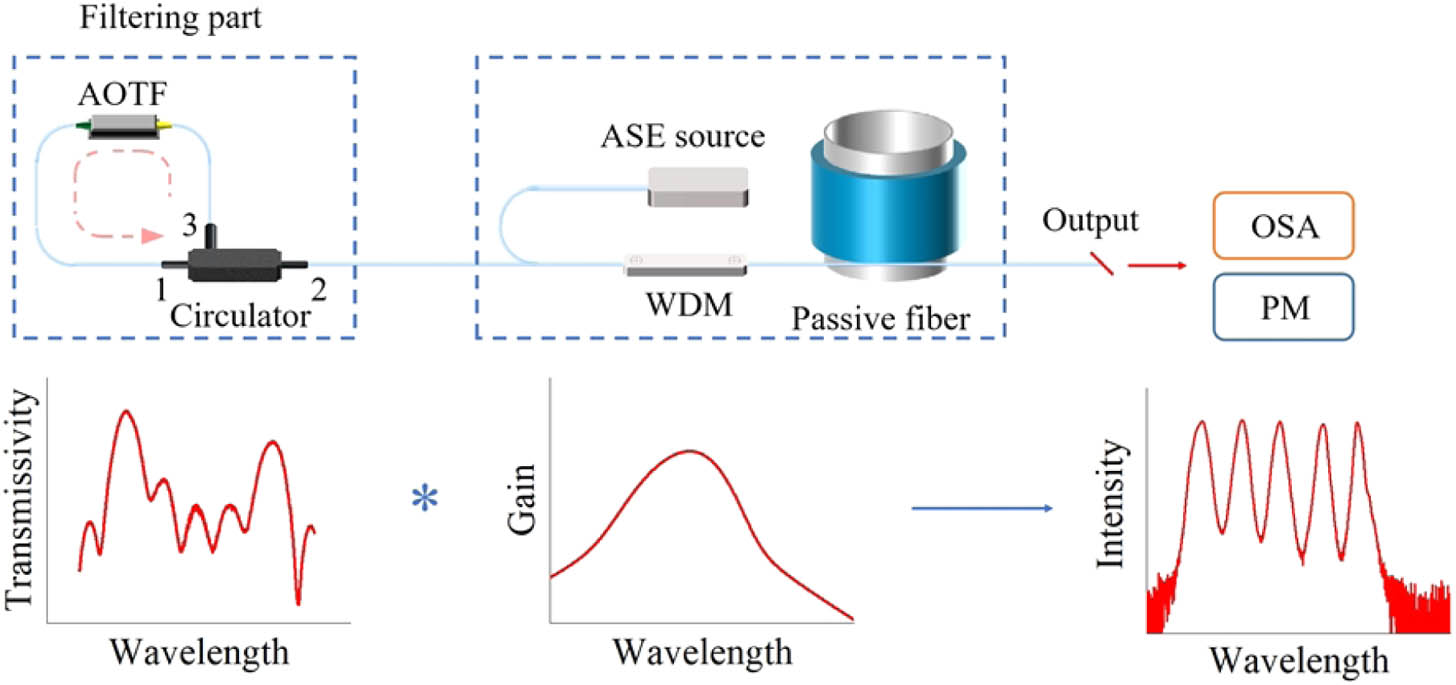
Set citation alerts for the article
Please enter your email address