Abstract
Since the discovery of hydride superconductors, a significant challenge has been to reduce the pressure required for their stabilization. In this context, we propose that alloying could be an effective strategy to achieve this. We focus on a series of alloyed hydrides with the AMH6 composition, which can be made via alloying A15 AH3 (A = Al or Ga) with M (M = a group ⅢB or IVB metal), and study their behavior under pressure. Seven of them are predicted to maintain the A15-type structure, similar to AH3 under pressure, providing a platform for studying the effects of alloying on the stability and superconductivity of AH3. Among these, the A15-type phases of AlZrH6 and AlHfH6 are found to be thermodynamically stable in the pressure ranges of 40–150 and 30–181 GPa, respectively. Furthermore, they remain dynamically stable at even lower pressures, as low as 13 GPa for AlZrH6 and 6 GPa for AlHfH6. These pressures are significantly lower than that required for stabilizing A15 AlH3. Additionally, the introduction of Zr or Hf increases the electronic density of states at the Fermi level compared with AlH3. This enhancement leads to higher critical temperatures (Tc) of 75 and 76 K for AlZrH6 and AlHfH6 at 20 and 10 GPa, respectively. In the case of GaMH6 alloys, where M represents Sc, Ti, Zr, or Hf, these metals reinforce the stability of the A15-type structure and reduce the lowest thermodynamically stable pressure for GaH3 from 160 GPa to 116, 95, 80, and 85 GPa, respectively. Particularly noteworthy are the A15-type GaMH6 alloys, which remain dynamically stable at low pressures of 97, 28, 5, and 6 GPa, simultaneously exhibiting high Tc of 88, 39, 70, and 49 K at 100, 35, 10, and 10 GPa, respectively. Overall, these findings enrich the family of A15-type superconductors and provide insights for the future exploration of high-temperature hydride superconductors that can be stabilized at lower pressures.I. INTRODUCTION
In recent years, hydrides have emerged as potential materials in the search of room-temperature superconductivity, not only from theoretical predictions1–5 but also from experimental verifications.6–22 In 2015, the predicted cubic H3S was synthesized for the first time and was experimentally confirmed to have a Tc of 203 K at 155 GPa.7,8 Four years later, the Tc record passed to the LaH10 metal hydride, with an H-clathrate structure, which was observed to have Tc values of 250–260 K at 170–190 GPa.9,10 Metallic clathrate hydrides were predicted to be a large class,23–28 and some of the predictions of superconductivity in this family have been successively verified experimentally, such as for ThH9/10 (146/161 K at 170–175 GPa),11 YH6 (224 K at 166 GPa and 220 K at 183 GPa),12,13 YH9 (243 K at 201 GPa and 262 K at 182 GPa),13,14 and CaH6 (215 K at 172 GPa and 210 K at 160 GPa).15,16 These achievements have greatly encouraged the exploration of room-temperature superconductivity in hydrides.
However, the possible application of superconducting hydrides is not only influenced by the superconducting transition temperature, as in the case of other superconductors, but also by the stabilization pressure. Although the hydrides mentioned above have high Tc values, the pressures required to stabilize them are also extremely high (>150 GPa). Therefore, it is essential to obtain superconducting hydrides that are stable at low pressures. Our previous study proposed that the introduction of light B into the La–H system should lead to a stable Fmm LaBH8 phase with BH8 units29–31 that remains dynamically stable at 55 GPa and exhibits a Tc of 155 K. Subsequently, some other B-, C-, and Si-based ternary hydrides were also estimated to show good superconductivity at moderate pressures.32–35 In these hydrides, the covalent units formed by including light elements with H contribute significantly to their low-pressure stability. Furthermore, an La–Y alloy tetrahydride was synthesized at 110 GPa, exhibiting a Tc of 92 K, and it can be recovered at 80 GPa, both of which are lower pressure thresholds than those required for the synthesis of YH4.18 Bi et al.19 synthesized an (La,Ce)H9 alloy at megabar pressures of 97–172 GPa, which exhibited a Tc of 148–178 K. More recently, the metastable compound P63/mmc-LaH10 was stabilized at 146 GPa by introducing Al atoms to form P63/mmc-(La,Al)H10, with a Tc of 178 K.20 An increase in the configurational entropy of a mixed alloy hydride will decrease its Gibbs free energy and enhance its stability. Therefore, alloying binary metal hydrides may be an alternative approach to optimize the stabilization pressure of superconducting hydrides.
Trihydrides are commonly found in binary metal hydrides, and their superconductivity under pressure has been extensively studied.36–45 On the basis of their structures, common metal trihydrides can be divided into two categories, one with the Pmn structure and the other with the Fmm structure. This provides a platform to tune the stability and superconductivity of hydrides by alloying. The Pmn structure, also called the A15 structure, is well known for its excellent superconducting performance. To date, about 50 alloys with this structure have been found to be superconductors, and some of them remain the most promising materials for practical applications.46 Among binary hydrides, AlH3, GaH3, ZrH3, and HfH3 were predicted to become stable in the A15 structure at 73, 160, 8, and 27 GPa, respectively, with the metal atoms forming a body-centered cubic (bcc) lattice and the six H atoms occupying half of the tetrahedral interstices of this lattice.36–40 Interestingly, the A15 phases of AlH3 and ZrH3 were confirmed in experiments at 100 and 30 GPa, respectively, but they do not exhibit high Tc values.37,39,41 On the other hand, the Al5 phase of GaH3 was estimated to have the highest Tc of 102 K at 120 GPa.38 However, A15 GaH3 requires relatively high minimum pressures of ∼160 and ∼84 GPa for thermodynamic and dynamic stability respectively,38,47 making its synthesis difficult. Below 160 GPa, A15 GaH3 becomes thermodynamically unstable and transforms into a structure containing H2 units, with an insulating character.26 Compared with Al and Ga, group IIIB and IVB metal atoms are less electronegative and could transfer more electrons to H2 units, allowing structures with atomic hydrogen to remain stable at lower pressures. In addition, group IIIB and IVB metals can also exist in the trivalent state and form trihydrides. Therefore, alloying AlH3/GaH3 with group IIIB/IVB metals could give stable alloy hydrides with an A15-type structure and the expected superconductivity at low pressures. For ternary hydrides, A15-type GaAsH6 and YZrH6 were predicted to have Tc values of 98 and 16 K at 180 GPa and ambient pressure, respectively.28,48 Recently, our group designed a series of A15-type ternary hydrides, with the CaSnH6 alloy demonstrating the lowest thermodynamically stable pressure of 110 GPa and CaSnH6 with the lowest dynamically stable pressure of 41 GPa,47 which also facilitated our exploration of ternary alloy hydrides. To some extent, alloying AlH3/GaH3 with group IIIB/IVB metals also provides a way to control the physical properties of ternary hydrides through the choice of their constituent elements.
In this work, we investigate theoretically the structure, stability, and superconductivity of alloy hydrides with the AMH6 composition (A = Al or Ga; M = a group IIIB or IVB metals). We chose the AMH6 composition because the substitution of an M atom for an A atom in the A2H6 unit cell is one of the most intuitive ways to form alloy hydrides. Furthermore, considering the promoting effect of configurational entropy in material formation, mixing metal elements in equal proportions may yield a highly disordered alloy hydride, making it more favorable for experimental synthesis. The results show that seven ternary hydrides, AlMH6 (M = Ti, Zr, or Hf) and GaMH6 (M = Sc, Ti, Zr, or Hf) are identified to be stable in the A15-type structure. A15-type AlZrH6, AlHfH6, and GaMH6 for all M are thermodynamically stable at pressures much lower than those required to stabilize A15 AlH3 and GaH3. Except for GaScH6, all of these hydrides also have significant advantages over AlH3 and GaH3 in terms of the lowest dynamically stable pressures, with AlZrH6, AlHfH6, GaZrH6, and GaHfH6 able to maintain their dynamical stability at ∼13, 6, 5, and 6 GPa, respectively. Electron–phonon coupling (EPC) calculations show that all of these ternary hydrides are superconducting. Among them, the estimated Tc values for AlZrH6, AlHfH6, GaScH6, GaZrH6, and GaHfH6 are 75, 76, 88, 70, and 49 K at 20, 10, 100, 10, and 10 GPa, respectively. In addition, AlScH6 and AlYH6 are predicted to be stable in structures similar to Fmm Sc(Y)H3, and they are also potential superconductors under pressure. Our results indicate that they have great potential for the exploration of low-pressure stable high-Tc superconductors in alloy hydrides.
II. COMPUTATIONAL DETAILS
Structure searches of AMH6 with simulation cells containing up to four formula units were performed at 50–300 GPa by using the particle swarm optimization technique implemented in the CALYPSO code.49,50 Structural relaxations and calculations of electronic properties were performed using the Vienna Ab Initio Simulation Package (VASP) code based on density functional theory (DFT) with the Perdew–Burke–Ernzerhof generalized gradient approximation.51,52 The ion–electron interaction was described by projector-augmented-wave potentials, with 1s1, 3s23p1, 3d104s24p1, 3s23p63d14s2, 4s24p64d15s2, 5s25p65d16s2, 3s23p63d24s2, 4s24p64d25s2, and 5p65d26s2 configurations being treated as valence electrons for H, Al, Ga, Sc, Y, La, Ti, Zr, and Hf atoms, respectively.53 The plane-wave kinetic energy cutoff was set to 700 eV, and the corresponding Monkhorst–Pack (MP) k-point meshes were adopted for different structures to ensure that enthalpy converged to 1 meV/atom. Phonon calculations were performed using the PHONOPY54 or Quantum-ESPRESSO codes.55 EPC calculations of Pm ternary hydrides were carried out with the Quantum-ESPRESSO code using ultrasoft pseudopotentials for all the atoms. A 9 × 9 × 9 q-point mesh in the first Brillouin zone (BZ) was used in the EPC calculation, and an MP grid of 36 × 36 × 36 was considered, to ensure k-point sampling convergence.
III. RESULTS AND DISCUSSION
We performed structure searches for 12 ternary hydrides with the AMH6 composition in the pressure range of 50–300 GPa. Seven hydrides (AlTiH6, AlZrH6, AlHfH6, GaScH6, GaTiH6, GaZrH6, and GaHfH6) were identified as having an A15-type structure with the Pm symmetry, as shown in Fig. 1(a). In this structure, two metal atoms occupy the vertex and the center positions to form a bcc lattice, with H atoms occupying locations near their tetrahedral interstices. The A–H, M–H, and H–H bond distances for Pm AMH6 at 100 GPa are shown in Table S1 (supplementary material). The A–H distance is shorter than the M–H distance in AlZrH6 and AlHfH6, while the reverse happens in AlTiH6 and GaMH6. Different metal atoms in the structure lead to two different lengths between adjacent H atoms. The calculated H–H distances of 1.45–1.75 Å are much longer than those of 0.74 and 1.2 Å in H2 and LaH10 at 100 GPa, respectively, indicating that H atoms are not bonded to each other. Except for GaTiH6 and GaScH6, the other five hydrides have no phase transition in the entire pressure range from 50 to 300 GPa. For GaTiH6 and GaScH6, the C2/m and Pmma structures, respectively, were predicted to be stable at 50 GPa, and they do not present H2 molecules in their structures (Fig. S1, supplementary material). Furthermore, AlScH6 and AlYH6 were predicted to have structures similar to Fmm Sc(Y)H3, where metal atoms form a face-centered cubic (fcc) lattice and H atoms are located at the tetrahedral and octahedral interstices of this lattice. As shown in Fig. S2 (supplementary material), Pmmn AlScH6 can be seen as a 2 × 2 × 1 supercell of Fmm Sc(Y)H3 with two metal atoms arranged alternately in two directions. P4/mmm AlScH6 is formed by replacing the two Sc atoms in Fmm ScH3 with Al atoms. I4/mmm AlYH6 is similar to P4/mmm AlScH6, but with slightly shifted H positions. The predicted structures of AlLaH6, GaYH6 and GaLaH6 are also shown in Fig. S3 (supplementary material).
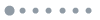
Figure 1.(a) Crystal structure of the A15-type ternary hydrides. Orange, magenta, and green balls represent A (Al or Ga), M (a group ⅢB or ⅣB metal), and H atoms, respectively. (b) Elements considered for the ternary hydrides. (c) Thermodynamic and (d) dynamical stability phase diagrams of the Pm hydrides with pressure.
Bader charge analyses56 were performed on A15-type ternary hydrides at 100 GPa, as shown in Table S2 (supplementary material). The results demonstrate the transfer of electronic charges from metal to H atoms, suggesting an ionic nature of the bonding between them. Each H atom in AlMH6 and GaMH6 accepts ∼0.60–0.64 and 0.39–0.43e, respectively. In AlMH6, Al atoms transfer around 2.31–2.71e to H atoms, surpassing the ∼1.31 to 1.54e transferred from the M atoms. In GaMH6, the M atom loses a greater amount of charge (∼1.34 to 1.61e) compared with the Ga atom (∼0.94 to 1.00e), indicating a greater ionic nature of the M–H bond. The electrons acquired by the H2 molecule occupy its antibonding orbitals, leading to an elongation of the H–H bond length and potentially even dissociation of the H2 unit. In AlMH6, the significant electron transfer from Al to H atoms compensates for the relatively smaller electrons transfer from the M atom. In contrast to GaH3, the doping M atoms enable H atoms to gain more charge in GaMH6 at the same pressure, thereby allowing these ternary hydrides to exhibit structures with atomic H at lower pressures. Additionally, the electron localization functions (ELFs) were calculated for these A15 ternary hydrides at 100 GPa with an isosurface value of 0.5, as depicted in Fig. S4 (supplementary material). The ELFs indicate that electrons are predominantly localized around the H atoms, confirming their role as electron acceptors. The ELF values below 0.5 between adjacent H atoms indicate the absence of H–H covalent bonds. The ELF values between the metal and H atoms approach zero, confirming their ionic bonding character, which is consistent with the aforementioned Bader charge analysis.
The thermodynamic stability of these ternary hydrides has been assessed by calculating their formation enthalpies relative to elemental solids and binary compounds.36,38–40,42–45 The relative enthalpy curves for AMH6 are presented in Fig. 2 and Fig. S5 (supplementary material). The calculations consider the most stable configuration for each component, and the total energy of AH3 and MH3 is used as the reference energy. Within certain pressure ranges, AMH6 exhibits lower formation enthalpy compared with possible decomposition pathways, indicating that AMH6 is thermodynamically stable. For AlMH6 (M = Ti, Zr, or Hf), the predicted A15-type phases remain stable within the pressure ranges of 78–165, 69–123, and 43–157 GPa, respectively. On accounting for zero-point energy (ZPE), the stable pressure range for AlTiH6 shrinks to 92–113 GPa and the relative formation enthalpy reduces to only −10 meV/f.u. Conversely, with ZPE corrections, the stable pressure ranges for AlZrH6 and AlHfH6 expand to 40–150 and 30–181 GPa, respectively. Furthermore, the stability pressure thresholds for both hydrides are lower than the 73 GPa threshold for AlH336 [Fig. 1(c)]. Regarding GaMH6 (M = Sc, Ti, Zr, or Hf), the predicted A15-type phases are stable above 126, 96, 85, and 95 GPa, respectively, and remain stable with increasing pressure. With ZPE taken into account, the minimal stable pressures change to 116, 95, 80, and 86 GPa, respectively, which are well below the stability threshold of 160 GPa for GaH3 [Fig. 1(c)]. Therefore, it is anticipated that the experimental synthesis of A15-type GaMH6 might be easier compared with that of GaH3. Inspired by the successful synthesis of the equal-atomic (La,Y)H4 and (La,Ce)H9 alloys, the high-temperature and high-pressure reaction of AM alloys with NH3BH3 might be a promising route for the synthesis of the alloys AMH6.18,19 Figure S5 (supplementary material) demonstrates that the Fmm-like AlScH6 is stable above 150 GPa and undergoes a transformation from the Pmmn phase to the P4/mmm phase at 318 GPa. The C2/m phase of AlYH6 is stable above 45 GPa and transforms to the Fmm-like I4/mmm phase at 115 GPa. AlLaH6 is predicted to exhibit the P63/mmc and Cmcm structures below ∼200 GPa. For GaYH6 and GaLaH6, the low-symmetry P21212 and C2/m structures are stable near 100 GPa and below 150 GPa, respectively. Additionally, we also performed structure predictions and first-principles calculations for hydrides with higher H content in the Al–Zr–H, Ga–Sc–H, Ga–Zr–H, and Ga–Hf–H systems at 200 and 300 GPa. As shown in Fig. S6 (supplementary material), these H-rich hydrides have higher formation enthalpies relative to AH3+ BH3 + H2 or AMH6 + H2, indicating that they are unstable at the corresponding pressures.
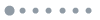
Figure 2.Relative enthalpy curves of predicted structures for (a) AlZrH6, (b) AlHfH6, (c) GaScH6, (d) GaTiH6, (e) GaZrH6, and (f) GaHfH6 with respect to AH3 (A = Al and Ga) and MH3 (M = Sc, Ti, Zr, and Hf) under pressure. The insets show the relative enthalpies with ZPE taken into account. The following structures for the elemental solids and binary hydrides were used for the ΔH calculations: P63/m, C2/c, and Cmca for H2; Fmm and P63/mmc for Al; Fmm for Ga; Rc, Pnma, and Pmn for AlH3; P21/m and Pmn for GaH3; Pmn and Rc for ZrH3; I3d for Zr4H15; Cmc21 and I4/mmm for ZrH6; Pmn for HfH3; I3d for Hf4H15; Cmc21 for HfH6; Fmm for ScH3; Cmcm, P63/mmc, and Imm for ScH6; Fmm for TiH3; I for Ti5H14; Fddd for TiH4.
To better understand why the A15-type AMH6 structure is stable, we conducted an analysis of the influence of the relative internal energy (ΔU) and the product of pressure and volume (ΔPV) on the relative enthalpy (ΔH) under pressure. The findings for AlZrH6 and AlHfH6 are illustrated in Fig. S7 (supplementary material). As pressure increases, the ΔPV term also rises and eventually exhibits positive values. Conversely, the ΔU term exhibits the opposite trend, indicating that the bonding in both ternary hydrides contributes significantly to their stabilization. For the A15-type GaMH6 (Fig. S8, supplementary material), the ΔPV contribution in all four hydrides is negative in comparison with the benchmark, indicating that the ΔPV term plays a dominant role in their thermodynamic stability. Meanwhile, the magnitude of ΔU decreases with increasing pressure, leading to an overall enhancement of the stability of these ternary hydrides. Furthermore, in GaScH6, the ΔPV and ΔU terms exhibit contrasting trends when compared with ScH3 + GaH3. The negative ΔPV contribution counteracts the negative effect of the ΔU term, ultimately resulting in the stabilization of GaScH6. Both the ΔPV and ΔU contribution in GaTiH6, GaZrH6, and GaHfH6 have favorable effects on thermodynamic stability compared with their respective binary hydrides.
We also investigated the dynamical stability of the predicted A15-type AMH6 compounds by calculating the phonon spectra using the supercell approach implemented in the PHONOPY code54 (Figs. S14 and S15, supplementary material). Within their respective thermodynamically stable pressure ranges, no imaginary frequencies were observed in the phonon spectra of these compounds, indicating their dynamical stability. Furthermore, we systematically explored the minimum pressure required for dynamical stability of these A15-type AMH6. As pressure decreases, phonon softening begins to occur, eventually leading to instability with the appearance of imaginary frequencies at certain q-wave vectors. We plotted the evolution of the frequency with pressure at the q-wave vector where the largest imaginary frequency occurs. As depicted in Figs. S9 and S10 (supplementary material), A15-type GaScH6 exhibited the highest critical pressure for dynamical stability, estimated to be around 97 GPa. GaTiH6 followed, with a critical pressure of ∼28 GPa. AlTiH6 and AlZrH6 were found to be dynamically stable at lower pressures of about 18 and 13 GPa, respectively. Interestingly, the critical pressures for dynamical stability of AlHfH6, GaZrH6, and GaHfH6 were considerably lower, at around 6, 5, and 6 GPa, respectively. Moreover, the threshold pressures for dynamical stability of the five ternary hydrides (GaTiH6, AlTiH6, AlHfH6, GaZrH6, and GaHfH6) were much lower compared with those of AH3 and MH3 [Figs. S11 and S13 (supplementary material) and Fig. 1(d)]. This suggests that these ternary hydrides could potentially be recovered at lower pressures, provided that the barriers preventing them from decomposing are sufficiently high. Of the Fmm-like ternary hydrides, Pmmn AlScH6, P4/mmm AlScH6, and I4/mmm AlYH6 could maintain their dynamical stability to 65, 139, and 43 GPa, respectively (Figs. S12 and S16, supplementary material).
The electronic properties of the predicted A15-type AMH6 compounds were investigated at different pressures, and the results are displayed in Fig. 3, as well as Figs. S17 and S18 (supplementary material). The calculated density of states (DOS) reveals the presence of electronic states at the Fermi energy level (Ef), indicating that these hydrides exhibit metallic behavior within the studied pressure range. The contributions of the metal and hydrogen atoms to the total DOS are also depicted in the figures. Figure 3(d) highlights a distinct difference between the DOS of GaScH6 and the other six hydrides composed of Al/Ga and group IVB metals. In GaScH6, the hydrogen atoms contribute significantly to the DOS at Ef, suggesting the potential for excellent superconducting properties. In the remaining six hydrides, the group IVB metals have one additional valence electron compared with Sc atoms, causing a shift of Ef to higher energies relative to GaScH6. These six hydrides exhibit higher or comparable total DOS at Ef compared with GaScH6. However, the DOS contributions from Al and Ga atoms are lower owing to significant charge transfer to the H atoms. In the case of the group IVB metals, the DOS at Ef is mainly contributed by d orbitals. Furthermore, the electronic band structures of AlHfH6, GaScH6, and GaZrH6 were examined as examples at pressures of 10, 100, and 10 GPa, respectively. The band projections onto different elements are also displayed in the band structures. In these three hydrides, a band associated with Al/Ga atoms is observed to cross Ef steeply along the M–R–Γ direction. Additionally, in AlHfH6 and GaZrH6 there is an electron pocket at the M point and a flat band along the Γ–M direction, dominated by Hf and Zr atoms, located near Ef. In the case of GaScH6, electron and hole pockets are observed along the X–M direction, along with a flat band along the Γ–M direction, which are attributed to H atoms. These localized electronic states contribute to a high DOS at the Fermi energy and play a role in electron–phonon interactions. Moreover, a comparison was made between the DOS of A15-type AlH3, AlTiH6, AlZrH6, and AlHfH6 at 100 GPa. As shown in Fig. S17 (supplementary material), the DOS at Ef for AlTiH6, AlZrH6, and AlHfH6 are 0.030, 0.018, and 0.018 states eV−1 Å−3 respectively, which are significantly higher than that of AlH3 (0.008 states eV−1 Å−3). The doping of AlH3 with Ti, Zr and Hf atoms elevated Ef, resulting in an increased DOS at Ef. Ternary hydrides exhibit a more pronounced metallic character compared with AlH3, potentially enhancing their superconducting properties.
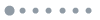
Figure 3.Calculated band structures and electronic DOS of Pm ternary hydrides at different pressures.
After determining the stability and metallicity of A15-type ternary hydrides, we conducted EPC calculations to investigate their superconductivity. The calculated phonon dispersion curves, projected phonon density of states (PDOS), Eliashberg phonon spectral function α2F(ω)/ω, and its integral λ(ω) for Pm AlHfH6, GaScH6 and GaZrH6 at pressures of 10, 100, and 10 GPa are displayed in Fig. 4. From the PDOS, it is evident that the high-frequency and low-frequency phonon modes are associated with the vibrations of H and metal atoms, respectively. The right-hand panels of Fig. 4 illustrate that the peaks of α2F(ω)/ω for AlHfH6, GaScH6, and GaZrH6 are predominantly distributed below 15, 20, and 15 THz, respectively, with the corresponding λ(ω) increasing rapidly. The EPC strength on the different phonon modes is also depicted on the phonon dispersions. As a result, the significant contribution to the EPC comes from the soft modes associated with H-atom vibrations and the modes dominated by metal atoms. This leads to a total EPC parameter λ of 2.18, 1.56, and 1.80 for AlHfH6, GaScH6, and GaZrH6 at pressures of 10, 100, and 10 GPa, respectively.
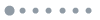
Figure 4.Calculated phonon dispersion curves (the area of the red circles is proportional to the EPC strength), projected phonon density of states (PDOS), Eliashberg phonon spectral function α2F(ω)/ω, and its integral λ(ω) for (a) Pm AlHfH6 at 10 GPa, (b) Pm GaScH6 at 100 GPa, and (c) Pm GaZrH6 at 10 GPa.
The superconducting critical temperatures of the predicted A15-type ternary hydrides were calculated using the Allen–Dynes modified McMillan equation with a Coulomb pseudopotential parameter μ* ranging from 0.1 to 0.13.57 The calculated Tc values, along with the EPC parameter λ and the phonon frequency logarithmic average ωlog are presented in Fig. 5 and Table I. For AlTiH6, the calculated EPC parameter λ is 1.15 and the phonon frequency logarithmic average ωlog is 423 K. This results in a Tc of 32–36 K at 20 GPa. AlZrH6 and AlHfH6 exhibit stronger EPC interactions, with λ of 1.72 and 2.18, and the Tc values are estimated to be 59–64 and 57–60 K at 20 and 10 GPa, respectively. Considering that the λ values are higher than 1.5, the calculated Tc values are further rectified with strong-coupling (f1) and shape (f2) corrections and improved to 68–75 and 70–76 K, respectively. The Tc values obtained for AlMH6 are indeed higher than those of AlH3 and the corresponding MH3 compounds.37,39,40,45 Similarly, GaScH6 exhibits the highest Tc among the studied hydrides, reaching a range of 79–88 K at a higher pressure of 100 GPa, However, its Tc is slightly lower than that of GaH3, which has a Tc of 102 K at 120 GPa. GaTiH6 has a lower Tc range of 34–39 K at 35 GPa compared with the Tc of 63–70 K for GaZrH6 and 45–49 K for GaHfH6 at 10 GPa.
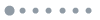
Figure 5.Estimated Tc of the predicted A15 AMH6 using the Allen–Dynes modified McMillan equation and those of the already known A(M)H3 compounds extracted from the literature.38–40,43–45
Phase | Pressure (GPa) | λ | ωlog | Tc (K), μ* = 0.1–0.13 | Tc (K) with f1 and f2, μ* = 0.1–0.13 |
---|
AlTiH6 | 20 | 1.15 | 423 | 32–36 | |
AlZrH6 | 20 | 1.72 | 494 | 59–64 | 68–75 |
| 30 | 1.18 | 667 | 52–59 | |
AlHfH6 | 10 | 2.18 | 397 | 57–60 | 70–76 |
| 20 | 1.28 | 596 | 52–58 | |
GaScH6 | 100 | 1.56 | 641 | 69–76 | 79–88 |
| 120 | 1.09 | 890 | 71–62 | |
GaTiH6 | 35 | 1.17 | 448 | 34–39 | |
| 100 | 0.68 | 887 | 29–22 | |
GaZrH6 | 10 | 1.80 | 438 | 54–58 | 63–70 |
| 80 | 0.81 | 898 | 43–35 | |
GaHfH6 | 10 | 1.42 | 454 | 45–49 | |
| 86 | 0.74 | 802 | 32–25 | |
Table 1. Calculated values of the EPC parameter λ, phonon frequency logarithmic average ωlog, critical temperature Tc (μ* = 0.1–0.13) using the Allen–Dynes modified McMillan equation without and with strong-coupling and shape corrections for Pm ternary hydrides.
The evolution of Tc with pressure was also investigated for Pm AMH6. For AlZrH6, the calculated EPC parameter λ decreased from 1.72 at 20 GPa to 1.18 at 30 GPa, while ωlog increased from 494 to 667 K, resulting in a slight decrease in Tc with μ* of 0.1 from 64 to 59 K (Allen–Dynes modified McMillan equation). Similarly, as the pressure increased from 10 to 20 GPa, the λ of AlHfH6 decreased from 2.18 to 1.28 and the ωlog increased from 397 to 596 K. As a result, the calculated Tc with μ* of 0.1 decreased slightly from 60 to 58 K. The increase in pressure leads to a stiffening of the phonon modes, which results in a decrease in the EPC parameter and an increase in ωlog. This compensating effect prevents a significant reduction in Tc. In addition, the Tc values of GaMH6 compounds decrease with increasing pressure, owing to the competition between the decreasing EPC parameter λ and the increasing phonon frequency logarithmic average ωlog. Of the Fmm-like ternary hydrides, Pmmn AlScH6, P4/mmm AlScH6, and I4/mmm AlYH6 are found to be metallic (Fig. S19, supplementary material), and subsequent EPC calculations suggest that they exhibit superconductivity. The Tc values of these hydrides are estimated to be 42, 32, and 52 K at pressures of 80, 200, and 60 GPa, respectively (Table S3, supplementary material).
IV. CONCLUSIONS
We have carried out crystal structural predictions and first-principles calculations on alloy hydrides with the AMH6 composition under pressure. Seven ternary hydrides, AlMH6 (M = Ti, Zr, or Hf) and GaMH6 (M = Sc, Ti, Zr, or Hf) were predicted to adopt the A15-type structure, and the calculated enthalpy curves indicated that the A15-type AlMH6 are stable within specific pressure ranges of 92–113, 40–150 and 30–181, respectively. The pressures for onset of stability for AlZrH6 and AlHfH6 are lower than the stability threshold of 73 GPa for AlH3. Phonon calculations demonstrated that A15-type AlTiH6, AlZrH6, and AlHfH6 can be dynamically stable at relatively low pressures, such as 18, 13, and 6 GPa, respectively. Additionally, the addition of Ti, Zr, and Hf into AlH3 influenced the position of the Fermi level, resulting in improved metallicity compared with AlH3. Consequently, the ternary hydrides exhibited higher Tc of 36, 75, and 76 K at 20, 20, and 10 GPa, respectively. The A15-type GaMH6 hydrides (GaScH6, GaTiH6, GaZrH6, and GaHfH6) were found to have minimum pressure thresholds for thermodynamic stability at 116, 95, 80, and 86 GPa, respectively, which are significantly lower than the stability threshold of 160 GPa for GaH3. Additionally, dynamical stability calculations indicated their potential retention down to lower pressures of 97, 28, 5, and 6 GPa, respectively. EPC calculations revealed that A15-type GaMH6 hydrides are superconducting, with Tc of 88, 39, 70, and 49 K at 100, 35, 10, and 10 GPa, respectively. AlScH6 and AlYH6 were predicted to be stable in Fmm Sc(Y)H3-like structures under pressure. The estimated Tc values for Pmmn, P4/mmm AlScH6, and I4/mmm AlYH6 were 42, 32, and 52 K at 80, 200, and 60 GPa, respectively. These findings suggest that alloying holds promise as a route to lower the stabilization pressure of hydrides, enabling the exploration of high-Tc hydride superconductors that can be stable at lower or ambient pressures.
SUPPLEMENTARY MATERIAL
ACKNOWLEDGMENTS
Acknowledgment. This work was supported by the Natural Science Foundation of China (Grant Nos. 52022089, 52372261, 52288102, and 11964026), the National Key R&D Program of China (Grant No. 2022YFA1402300), the Natural Science Foundation of Hebei Province (Grant No. E2022203109), the Doctoral Fund of Henan University of Technology (Grant No. 31401579). P.L. thanks the Science and Technology Leading Talents and Innovation Team Building Projects of the Inner Mongolia Autonomous Region (Grant No. GXKY22060). A.B. acknowledges financial support from the Spanish Ministry of Science and Innovation (Grant No. FIS2019-105488GB-I00) and the Department of Education, Universities and Research of the Basque Government and the University of the Basque Country (Grant No. IT1707-22). E.Z. acknowledges the National Science Foundation (Grant No. DMR-2136038) for financial support.
References
[1] G.Profeta, A.Sanna, L.Boeri, M.Eremets, J. A.Flores-Livas, R.Arita. A perspective on conventional high-temperature superconductors at high pressure: Methods and materials. Phys. Rep., 856, 1(2020).
[2] A. G.Kvashnin, A. R.Oganov, I. A.Savkin, I. A.Kruglov, D. V.Semenok. On distribution of superconductivity in metal hydrides. Curr. Opin. Solid State Mater. Sci., 24, 100808(2020).
[3] H.Wang, X.Li, Y.Ma, G.Gao, Y.Li. Hydrogen-rich superconductors at high pressures. Wiley Interdiscip. Rev.: Comput. Mol. Sci., 8, e1330(2018).
[4] K. P.Hilleke, E.Zurek. Tuning chemical precompression: Theoretical design and crystal chemistry of novel hydrides in the quest for warm and light superconductivity at ambient pressures. J. Appl. Phys., 131, 070901(2022).
[5] V. V.Struzhkin, R. T.Howie, J.Zhang, G.Gao, M.Li, L.Wang, E.Gregoryanz, L.Wang, J. S.Tse. Superconducting binary hydrides: Theoretical predictions and experimental progresses. Mater. Today Phys., 21, 100546(2021).
[6] S. L.Bud’ko, D.Sun, P. P.Kong, F. F.Balakirev, A. P.Drozdov, R.Prozorov, V.Ksenofontov, M. I.Eremets, S. I.Shylin, V. S.Minkovet?al.. High-temperature superconductivity in hydrides: Experimental evidence and details. J Supercond. Nov. Magn., 35, 965(2022).
[7] A. P.Drozdov, S. I.Shylin, V.Ksenofontov, I. A.Troyan, M. I.Eremets. Conventional superconductivity at 203 kelvin at high pressures in the sulfur hydride system. Nature, 525, 73(2015).
[8] A. P.Drozdov, N.Hirao, K.Shimizu, M. I.Eremets, I. A.Troyan, M.Einaga, T.Ishikawa, Y.Ohishi, M.Sakata. Crystal structure of the superconducting phase of sulfur hydride. Nat. Phys., 12, 835(2016).
[9] M. A.Kuzovnikov, L.Balicas, S. P.Besedin, F. F.Balakirev, V. S.Minkov, D. e.Graf, P. P.Kong, V. B.Prakapenka, S.Mozaffari, A. P.Drozdovet?al.. Superconductivity at 250 K in lanthanum hydride under high pressures. Nature, 569, 528(2019).
[10] R. J.Hemley, V. V.Struzhkin, Y.Meng, Z. M.Geballe, M.Ahart, A. K.Mishra, M.Somayazulu, M.Baldini. Evidence for superconductivity above 260 K in lanthanum superhydride at megabar pressures. Phys. Rev. Lett., 122, 027001(2019).
[11] O. A.Sobolevskiy, V.Svitlyk, V. Yu.Fominski, A. V.Sadakov, I. A.Troyan, D. V.Semenok, A. R.Oganov, A. G.Ivanova, A. G.Kvashnin, V. M.Pudalov. Superconductivity at 161 K in thorium hydride ThH10: Synthesis and properties. Mater. Today, 33, 36(2020).
[12] V. M.Pudalov, A. G.Kvashnin, I. A.Troyan, V. B.Prakapenka, O. A.Sobolevskiy, E.Greenberg, A. G.Ivanova, A. G.Gavriliuk, D. V.Semenok, A. V.Sadakovet?al.. Anomalous high-temperature superconductivity in YH6. Adv. Mater., 33, 2006832(2021).
[13] S.Mozaffari, V. B.Prakapenka, S. P.Besedin, M. A.Kuzovnikov, P.Kong, F. F.Balakirev, S.Chariton, A. P.Drozdov, V. S.Minkov, L.Balicaset?al.. Superconductivity up to 243 K in the yttrium-hydrogen system under high pressure. Nat. Commun., 12, 5075(2021).
[14] R.McBride, A.Salamat, E.Zurek, K. V.Lawler, X.Wang, R. P.Dias, N.Meyers, N.Dasenbrock-Gammon, E.Snider. Synthesis of yttrium superhydride superconductor with a transition temperature up to 262 K by catalytic hydrogenation at high pressures. Phys. Rev. Lett., 126, 117003(2021).
[15] H.Wang, X.Yang, Y.Zhao, Y.Wang, H.Liu, M.Zhou, Y.Xie, K.Wang, L.Ma, X.Yuet?al.. High-temperature superconducting phase in clathrate calcium hydride CaH6 up to 215 K at a pressure of 172 GPa. Phys. Rev. Lett., 128, 167001(2022).
[16] Y.Jia, Z.Li, J.Zhang, S.Feng, K.Lu, S.Zhang, C.Zhang, X.He, J.Zhao, X.Wanget?al.. Superconductivity above 200 K discovered in superhydrides of calcium. Nat. Commun., 13, 2863(2022).
[17] A. G.Ivanova, I. A.Kruglov, I. S.Lyubutin, K. S.Pervakov, M.Hanfland, I. A.Troyan, A. V.Sadakov, D. V.Semenok, O. A.Sobolevskiy, A. G.Kvashninet?al.. Superconductivity at 253 K in lanthanum–yttrium ternary hydrides. Mater. Today, 48, 18(2021).
[18] Y.Wang, P.Zhang, K.Shimizu, J.Bi, Y.Nakamoto, Y.Wang, L.Ma, H.Liu, M.Zhou, B.Zouet?al.. Stabilization of superconductive La–Y alloy superhydride with Tc above 90 K at megabar pressure. Mater. Today Phys., 28, 100840(2022).
[19] G.Liu, P.Zhang, B.Zou, Y.Nakamoto, M.Zhou, J.Bi, Y.Ma, H.Wang, K.Shimizu, H.Liu. Giant enhancement of superconducting critical temperature in substitutional alloy (La,Ce)H9. Nat. Commun., 13, 5952(2022).
[20] H.Yuan, T.Cui, J.Guo, J.Zhang, W.Chen, K.Zhang, Y.Qian, X.Huang, S.Chen. High-temperature superconductivity up to 223 K in the Al stabilized metastable hexagonal lanthanum superhydride. Natl. Sci. Rev(2023).
[21] Y.Huang, H.Xie, B.Liu, X.Li, D.Duan, Q.Zhou, C. J.Pickard, X.Huang, D.Zhou, Q.Zhuang, T.Cui. Polyhydride CeH9 with an atomic-like hydrogen clathrate structure. Nat. Commun., 10, 3461(2019).
[22] N. P.Salke, I. A.Kruglov, J.-F.Lin, J.Zhou, M. M.Davari Esfahani, A. R.Oganov, Y.Zhang, J.Liu, V. B.Prakapenka, E.Greenberg, Y.Wang. Synthesis of clathrate cerium superhydride CeH9 at 80-100 GPa with atomic hydrogen sublattice. Nat. Commun., 10, 4453(2019).
[23] J. S.Tse, K.Tanaka, Y.Ma, T.Iitaka, H.Wang. Superconductive sodalite-like clathrate calcium hydride at high pressures. Proc. Natl. Acad. Sci. U. S. A., 109, 6463(2012).
[24] Y.Ma, H.Liu, Y.Wang, J. S.Tse, J.Hao, Y.Li. Pressure-stabilized superconductive yttrium hydrides. Sci. Rep., 5, 9948(2015).
[25] N. W.Ashcroft, R. J.Hemley, H.Liu, I. I.Naumov, R.Hoffmann. Potential high- Tc superconducting lanthanum and yttrium hydrides at high pressure. Proc. Natl. Acad. Sci. U. S. A., 114, 6990(2017).
[26] Y.Ma, Q.Wu, Y.Sun, R. J.Needs, F.Peng, C. J.Pickard. Hydrogen clathrate structures in rare earth hydrides at high pressures: Possible route to room-temperature superconductivity. Phys. Rev. Lett., 119, 107001(2017).
[27] B.Wen, X.-F.Zhou, J.He, X.Liang, Z.Zhao, A.Bergara, Y.Tian, L.Wang, G.Gao. Potential high-Tc superconductivity in CaYH12 under pressure. Phys. Rev. B, 99, 100505(2019).
[28] T.Ishikawa, T.Miyake, K.Shimizu. Materials informatics based on evolutionary algorithms: Application to search for superconducting hydrogen compounds. Phys. Rev. B, 100, 174506(2019).
[29] X.Song, H.Liu, X.Liang, L.Wang, Y.Tian, A.Bergara, R. J.Hemley, X.Wei, L.Wang, G.Gao, R.Sun. Prediction of high-Tc superconductivity in ternary lanthanum borohydrides. Phys. Rev. B, 104, 134501(2021).
[30] M. J.Hutcheon, A. M.Shipley, C. J.Pickard, Z.Zhang, Y.Yao, HM.SongDu, T.Cui, D.Duan, V. Z.Kresin. Design principles for high-temperature superconductors with a hydrogen-based alloy backbone at moderate pressure. Phys. Rev. Lett., 128, 047001(2022).
[31] C.Heil, L.Boeri, W.von der Linden, S.Di Cataldo. LaBH8: Towards high-Tc low-pressure superconductivity in ternary superhydrides. Phys. Rev. B, 104, L020511(2021).
[32] W.von der Linden, R.Lucrezi, C.Heil, S.Di Cataldo, L.Boeri. In-silico synthesis of lowest-pressure high-Tc ternary superhydrides. npj Comput. Mater., 8, 119(2022).
[33] H.Liu, Y.Sun, X.Zhong, S.Sun. Prediction for high superconducting ternary hydrides below megabar pressure. J. Phys.: Condens. Matter, 34, 505404(2022).
[34] M.Gao, X.-W.Yan, T.Xiang, Z.-Y.Lu. Phonon-mediated high-temperature superconductivity in the ternary borohydride KB2H8 under pressure near 12 GPa. Phys. Rev. B, 104, L100504(2021).
[35] Y.-L.Hai, G.-H.Zhong, H.-B.Ding, X.-J.Chen, M.-J.Jiang, H.-L.Tian, Y.-J.Feng, C.-L.Yang. High-temperature superconductivity below 100 GPa in ternary C-based hydride MC2H8 with molecular crystal characteristics (M=Na, K, Mg, Al, and Ga). Phys. Rev. B, 105, 104511(2022).
[36] R. J.Needs, C. J.Pickard. Metallization of aluminum hydride at high pressures: A first-principles study. Phys. Rev. B, 76, 144114(2007).
[37] M.Amboage, M. I.Eremets, I.Goncharenko, I. A.Trojan, J. S.Tse, M.Hanfland, Y.Yao. Pressure-induced hydrogen-dominant metallic state in aluminum hydride. Phys. Rev. Lett., 100, 045504(2008).
[38] G.Liu, Y.Ma, G.Gao, Y.Li, A.Bergara, H.Wang. Metallic and superconducting gallane under high pressure. Phys. Rev. B, 84, 064118(2011).
[39] H.Xie, T.Cui, X.Huang, Y.Huang, W.Zhang, X.Feng, H.Song, D.Duan, C. J.Pickard, Y.Yao. Superconducting zirconium polyhydrides at moderate pressures. J. Phys. Chem. Lett., 11, 646(2020).
[40] X.Feng, T.Cui, C. J.Pickard, H.Song, Y.Yao, H.Xie, V. Z.Kresin, S. A. T.Redfern, D.Duan, S.Jiang, Z.Zhang. Hydrogen pentagraphenelike structure stabilized by hafnium: A high-temperature conventional superconductor. Phys. Rev. Lett., 125, 217001(2020).
[41] B.Rousseau, A.Bergara. Giant anharmonicity suppresses superconductivity in AlH3 under pressure. Phys. Rev. B, 82, 104504(2010).
[42] X.Ye, R.Hoffmann, N. W.Ashcroft. Theoretical study of phase separation of scandium hydrides under high pressure. J. Phys. Chem. C, 119, 5614(2015).
[43] D. Y.Kim, T. W.Kang, H.-k.Mao, R. H.Scheicher, R.Ahuja. General trend for pressurized superconducting hydrogen-dense materials. Proc. Natl. Acad. Sci. U. S. A., 107, 2793(2010).
[44] R.Ahuja, D. Y.Kim, R. H.Scheicher. Predicted high-temperature superconducting state in the hydrogen-dense transition-metal hydride YH3 at 40 K and 17.7 GPa. Phys. Rev. Lett., 103, 077002(2009).
[45] X.Dong, J. M.McMahon, J.Zhang, S.Wang, H.Dong, X.Li, A. R.Oganov. High-temperature superconductivity in the Ti-H system at high pressures. Phys. Rev. B, 101, 134108(2020).
[46] G. R.Stewart. Superconductivity in the A15 structure. Physica C, 514, 28(2015).
[47] X.Liang, X.Song, P.Li, G.Gao, E.Zurek, L.Wang, A.Bergara, L.Wang, Y.Tian, X. X.WeiHao. Designing ternary superconducting hydrides with A15-type structure at moderate pressures. Mater. Today Phys., 34, 101086(2023).
[48] W.Zhao, Q.Jiang, D.Duan, T.Ma, M.Xu, H.Song, T.Cui, M.Du. Pressure-induced high-temperature superconductivity in ternary Y–Zr–H compounds. Phys. Chem. Chem. Phys., 25, 5237(2023).
[49] L.Zhu, J.Lv, Y.Wang, Y.Ma. Crystal structure prediction via particle-swarm optimization. Phys. Rev. B, 82, 094116(2010).
[50] Y.Ma, L.Zhu, J.Lv, Y.Wang. CALYPSO: A method for crystal structure prediction. Comput. Phys. Commun., 183, 2063(2012).
[51] J.Furthmüller, G.Kresse. Efficient iterative schemes for ab initio total-energy calculations using a plane-wave basis set. Phys. Rev. B, 54, 11169(1996).
[52] J. P.Perdew, K.Burke, M.Ernzerhof. Generalized gradient approximation made simple. Phys. Rev. Lett., 77, 3865(1996).
[53] P. E.Bl?chl. Projector augmented-wave method. Phys. Rev. B, 50, 17953(1994).
[54] I.Tanaka, A.Togo, F.Oba. First-principles calculations of the ferroelastic transition between rutile-type and CaCl2-type SiO2 at high pressures. Phys. Rev. B, 78, 134106(2008).
[55] S.Baroni, P.Giannozzi, C.Cavazzoni, M.Calandra, G.Fratesi, S.Fabris, R.Car, R. M.Wentzcovitch, L.Paulatto, S.de Gironcoli, M.Cococcioni, S.Paolini, I.Dabo, F.Mauri, G. L.Chiarotti, N.Bonini, C.Sbraccia, P.Umari, A.Dal Corso, D.Ceresoli, R.Gebauer, N.Marzari, R.Mazzarello, L.Martin-Samos, G.Sclauzero, U.Gerstmann, A.Pasquarello, A.Smogunov, C.Gougoussis, S.Scandolo, A. P.Seitsonen, M.Lazzeri, A.Kokalj. QUANTUM ESPRESSO: A modular and open-source software project for quantum simulations of materials. J. Phys.: Condens. Matter, 21, 395502(2009).
[56] R.Bader. Atoms in Molecules: A Quantum Theory(1994).
[57] R. C.Dynes, P. B.Allen. Transition temperature of strong-coupled superconductors reanalyzed. Phys. Rev. B, 12, 905(1975).