
- Photonics Research
- Vol. 9, Issue 4, 605 (2021)
Abstract
1. INTRODUCTION
Silicon photonics is promising for the demands of large-capacity data communications applications such as data centers, high-performance computers, and biomedical sensing [1,2]. One of the indispensable technologies in silicon photonics is high-speed signal detection for the near-infrared (
In this work, we propose and demonstrate a high-speed Ge vertical PIN PD, by comprehensively optimizing the parasitic parameters from both junction and electrodes. An equivalent circuit model containing transit time and all parasitic parameters is considered. The bandwidth limit under a given intrinsic region size is investigated through two-dimensional (2D) joint optimization, by varying the silicon doping concentration and electrodes inductance. Experimentally, the bandwidth is enhanced from 27 to 80 GHz, with a low dark current of 6.4 nA and an optical responsivity of 0.89 A/W, leading to a high detectivity. This is, to the best of our knowledge, the highest bandwidth of a CMOS-compatible vertical Ge PIN PD reported to date. This work provides guidance for the design of high-speed and high-detectivity Ge PDs.
2. OPERATION PRINCIPLE
Figure 1.Cross-section view and equivalent circuit for a conventional PD.
The normalized frequency response of the radio frequency (RF) current through the load is shown in
Sign up for Photonics Research TOC. Get the latest issue of Photonics Research delivered right to you!Sign up now
Figure 2.(a) Simulated bandwidth varying with
As an implementation, the series resistance
3. FABRICATION AND EXPERIMENTAL RESULTS
Figure 3.(a) 3D schematic of the PD-A. (b) Cross-section of the PD-A with full silicon
The PDs are fabricated using an SOI wafer with an 220 nm thick silicon top layer and a 2 μm buried oxide (BOX). The grating coupler is first etched 70 nm from 220 nm silicon layer. After that, the silicon layer is etched into a ridge waveguide with a 90 nm slab for better light constraints. Then, the silicon top layer is
A. Static Measurements
Figure 4.
B. Small-Signal Measurements
Small-signal RF measurements are carried out using a 67 GHz vector network analyzer (VNA: MS4647B, Anritsu Corp., Kanagawa, Japan) in the test range of 10 MHz to 70 GHz with 50 Ω load resistance. The impedance standard substrate [101-190C, FormFactor (formerly Cascade Microtech), Beaverton, OR, USA] is used to calibrate the bias-tee, cables, and microprobe (I67-GSG-150, FormFactor).
Figure 5.Measured and fitted bandwidth characteristics of Ge PDs.
4. DISCUSSION
To fairly characterize the proposed PDs, we take advantage of the concept of detectivity (
Comparison of the High-Speed Waveguide-Coupled Ge PDs
Refs. | Type | Dark Current (nA) | Responsivity (A/W) | Bandwidth (GHz) | Detectivity ( |
---|---|---|---|---|---|
[ | Vertical | 19 | 0.6 | 50 | 2.56 × 109 |
[ | Vertical | 3000 | 0.75 | 60 | 6.85 × 108 |
[ | Vertical | 61 | 0.85 | 67 | 4.30 × 109 |
[ | Lateral | 4000 | 0.8 | 120 | 8.94 × 108 |
[ | Lateral | 2.5 | 0.72 | 67 | 6.78 × 109 |
[ | Lateral | 40 | 0.5 | 50 | 3.13 × 109 |
This work | Vertical | 6.4 | 0.89 | 80 | 1.33 × 1010 |
5. CONCLUSION
We have demonstrated that 2D parasitic parameter engineering enables a significant bandwidth boost for Ge PDs. The bandwidth is experimentally enhanced from 27 to 80 GHz, and approaches the limit of 82 GHz. This method does not cause a degradation in responsivity and the dark current, which results in a very high detectivity. We believe it will pave the way toward the design of high-speed, high-detectivity Ge PDs.
References
[1] A. H. Atabaki, S. Moazeni, F. Pavanello, H. Gevorgyan, J. Notaros, L. Alloatti, M. T. Wade, C. Sun, S. A. Kruger, H. Y. Meng, K. Al Qubaisi, I. Wang, B. H. Zhang, A. Khilo, C. V. Baiocco, M. A. Popovic, V. M. Stojanovic, R. J. Ram. Integrating photonics with silicon nanoelectronics for the next generation of systems on a chip. Nature, 556, 349-354(2018).
[2] J. You, Y. K. Luo, J. Yang, J. H. Zhang, K. Yin, K. Wei, X. Zheng, T. Jiang. Hybrid/integrated silicon photonics based on 2D materials in optical communication nanosystems. Laser Photon. Rev., 14, 2000239(2020).
[3] J. Michel, J. F. Liu, L. C. Kimerling. High-performance Ge-on-Si photodetectors. Nat. Photonics, 4, 527-534(2010).
[4] H. T. Chen, P. Verheyen, P. De Heyn, G. Lepage, J. De Coster, P. Absil, G. Roelkens, J. Van Campenhout. High-responsivity low-voltage 28-Gb/s Ge p-i-n photodetector with silicon contacts. J. Lightwave Technol., 33, 820-824(2015).
[5] A. Novack, M. Gould, Y. S. Yang, Z. Xuan, M. Streshinsky, Y. Liu, G. Capellini, A. E. J. Lim, G. Q. Lo, T. Baehr-Jones, M. Hochberg. Germanium photodetector with 60 GHz bandwidth using inductive gain peaking. Opt. Express, 21, 28387-28393(2013).
[6] G. Y. Chen, Y. Yu, S. P. Deng, L. Liu, X. L. Zhang. Bandwidth improvement for germanium photodetector using wire bonding technology. Opt. Express, 23, 25700-25706(2015).
[7] L. Vivien, A. Polzer, D. Marris-Morini, J. Osmond, J. M. Hartmann, P. Crozat, E. Cassan, C. Kopp, H. Zimmermann, J. M. Fedeli. Zero-bias 40 Gbit/s germanium waveguide photodetector on silicon. Opt. Express, 20, 1096-1101(2012).
[8] H. Chen, P. Verheyen, P. De Heyn, G. Lepage, J. De Coster, S. Balakrishnan, P. Absil, W. Yao, L. Shen, G. Roelkens, J. Van Campenhout. −1 V bias 67 GHz bandwidth Si-contacted germanium waveguide p-i-n photodetector for optical links at 56 Gbps and beyond. Opt. Express, 24, 4622-4631(2016).
[9] L. Virot, D. Benedikovic, B. Szelag, C. Alonso-Ramos, B. Karakus, J. M. Hartmann, X. Le Roux, P. Crozat, E. Cassan, D. Marris-Morini, C. Baudot, F. Boeuf, J. M. Fedeli, C. Kopp, L. Vivien. Integrated waveguide PIN photodiodes exploiting lateral Si/Ge/Si heterojunction. Opt. Express, 25, 19487-19496(2017).
[10] J. F. Song, A. L. Eu-Jin, X. S. Luo, Y. Huang, X. G. Tu, L. X. Jia, Q. Fang, T. Y. Liow, M. B. Yu, G. Q. Lo. Microring resonator photodetector for enhancement in L-band performance. Opt. Express, 22, 26976-26984(2014).
[11] C. T. DeRose, D. C. Trotter, W. A. Zortman, A. L. Starbuck, M. Fisher, M. R. Watts, P. S. Davids. Ultra compact 45 GHz CMOS compatible germanium waveguide photodiode with low dark current. Opt. Express, 19, 24897-24904(2011).
[12] S. R. Liao, N. N. Feng, D. Z. Feng, P. Dong, R. Shafiiha, C. C. Kung, H. Liang, W. Qian, Y. Liu, J. Fong, J. E. Cunningham, Y. Luo, M. Asghari. 36 GHz submicron silicon waveguide germanium photodetector. Opt. Express, 19, 10967-10972(2011).
[13] L. Vivien, J. Osmond, J. M. Fedeli, D. Marris-Morini, P. Crozat, J. F. Damlencourt, E. Cassan, Y. Lecunff, S. Laval. 42 GHz p.i.n germanium photodetector integrated in a silicon-on-insulator waveguide. Opt. Express, 17, 6252-6257(2009).
[14] S. Lischke, D. Knoll, C. Mai, L. Zimmermann, A. Peczek, M. Kroh, A. Trusch, E. Krune, K. Voigt, A. Mai. High bandwidth, high responsivity waveguide-coupled germanium p-i-n photodiode. Opt. Express, 23, 27213-27220(2015).
[15] R. Going, T. J. Seok, J. Loo, K. Hsu, M. C. Wu. Germanium wrap-around photodetectors on silicon photonics. Opt. Express, 23, 11975-11984(2015).
[16] X. L. Li, Z. Liu, L. Z. Peng, X. Q. Liu, N. Wang, Y. Zhao, J. Zheng, Y. H. Zuo, C. L. Xue, B. W. Cheng. High-performance germanium waveguide photodetectors on silicon. Chin. Phys. Lett., 37, 038502(2020).
[17] T. Yin, R. Cohen, M. M. Morse, G. Sarid, Y. Chetrit, D. Rubin, M. J. Paniccia. 31 GHz Ge
[18] J. M. Lee, M. Y. Kim, W. Y. Choi. Series resistance influence on performance of waveguide-type germanium photodetectors on silicon. Chin. Opt. Lett., 15, 100401(2017).
[19] G. Wang, T. Tokumitsu, I. Hanawa, K. Sato, M. Kobayashi. Analysis of high speed pin photodiode S-parameters by a novel small-signal equivalent circuit model. IEEE Microw. Wireless Compon. Lett., 12, 378-380(2002).
[20] Y. Ishikawa, K. Wada. Near-infrared Ge photodiodes for Si photonics: operation frequency and an approach for the future. IEEE Photon. J., 2, 306-320(2010).
[21] M. Gould, T. Baehr-Jones, R. Ding, M. Hochberg. Bandwidth enhancement of waveguide-coupled photodetectors with inductive gain peaking. Opt. Express, 20, 7101-7111(2012).
[22] S. M. Sze, K. K. Ng. Physics of Semiconductor Devices(2006).
[23] J. Isenberg, W. Warta. Free carrier absorption in heavily doped silicon layers. Appl. Phys. Lett., 84, 2265-2267(2004).
[24] M. J. Deen, P. K. Basu. Silicon Photonics: Fundamentals and Devices(2012).
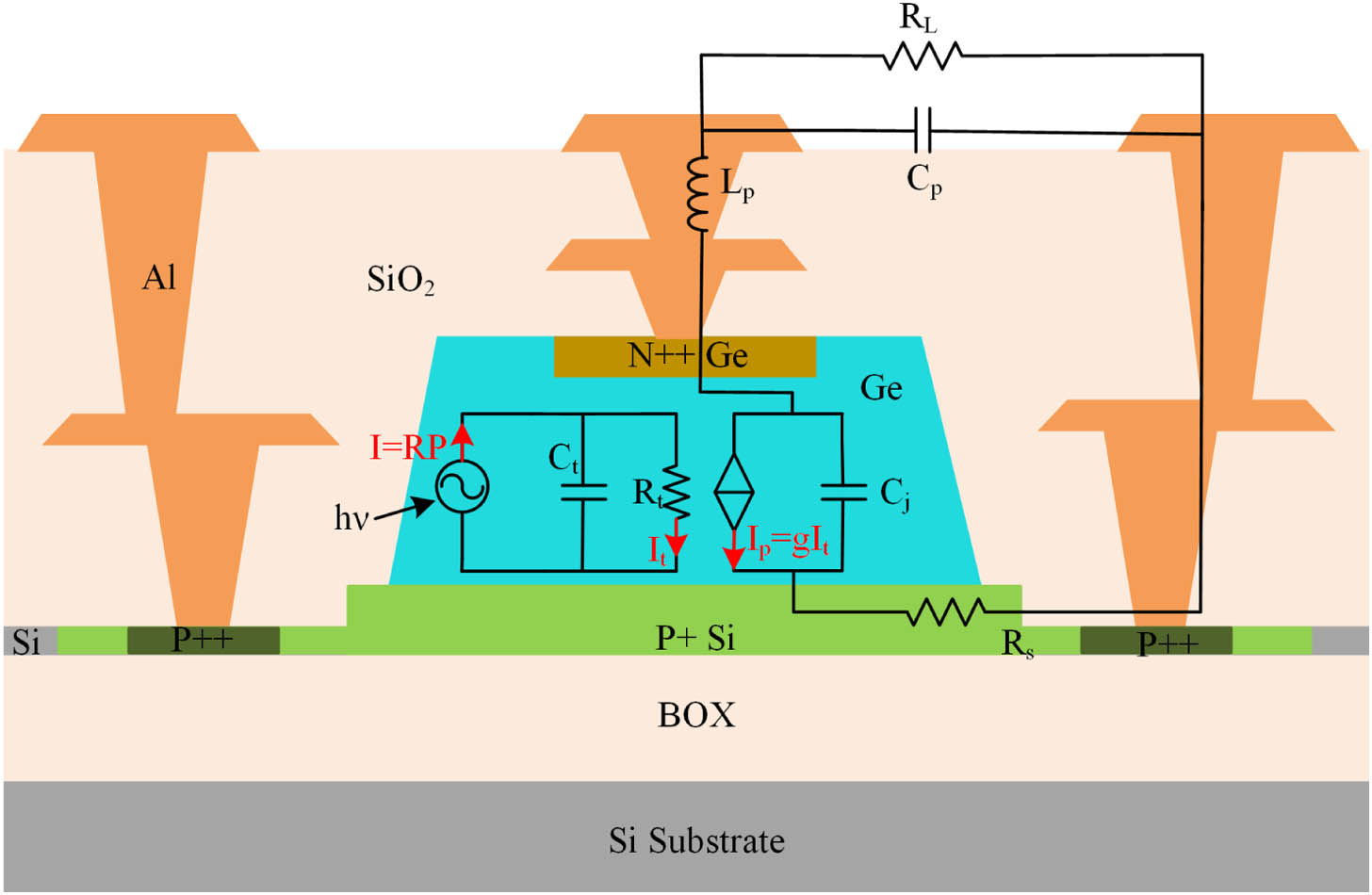
Set citation alerts for the article
Please enter your email address