
- Photonics Research
- Vol. 9, Issue 7, 1272 (2021)
Abstract
1. INTRODUCTION
High-efficient optical nonlinear processes based on high-quality () factor microresonators (MRs) have attracted huge attention for applications such as high-brightness and broadband lasing [1], coherent telecommunication [2], quantum optics [3], and high-precision sensing [4]. High- optical microresonators can feature high field confinement, long photon lifetime, and small mode volumes and therefore can dramatically boost the light–matter interaction within the resonators and reduce the power threshold [5–7]. Among others, chalcogenide glass (ChG) is an attractive material for the study of nonlinear optics because it has broad transparency from the visible to the footprint infrared region (up to ca. 20 μm), high Kerr nonlinearity (10–100 times higher than several typical amorphous materials), low two-photon absorption (TPA), and no free-carrier effects [8–10]. More importantly, being amorphous, ChG can adhere to different substrates by thermal evaporation at the low glass transition temperature (, less than 300°C) without surface modification or bonding process, which is compatible with a back-end CMOS process [9,11,12]. These advantages make ChGs versatile in numerous applications, including stimulated Brillouin scattering (SBS) [13], SBS oscillation [12,14,15], broadband supercontinuum generation [10], midinfrared parametric amplification [16], Raman amplification [17], and so on. Especially, optical frequency combs based on the optical parametric oscillation (OPO) process can now be implemented on a chip to revolutionize applications in metrology and sensing through optical parametric processes [18,19]. Of particular interest is to implement chip-scale and high- microresonators using ChG, which can induce intensive parametric oscillations at low power and can lead to the generation of optical Kerr frequency combs in operational wavelength windows including the midinfrared [12].
However, ChGs are believed to be unstable and can be easily oxidized. Previously reported ChG MRs usually showed a high level of thermal and light-induced instabilities, which decrease the robustness and efficiency of intracavity OPO [20]. In particular, as-deposited ChG films prepared by thermal evaporation typically contain a lot of homopolar bonds, voids, and subphases compared with bulk glasses. For instance, homopolar As-As bonds in ChG film will easily break and be reconstructed with oxygen when heated or being exposed to lasers in milliwatts [20]. The transformation of such bonds will then lead to the change of the refractive index (RI) and reduce stability of ChG film, which is detrimental to OPO [20–22]. As a solution, the thermal annealing process at a temperature close to has been used to promote the conversion of homopolar bonds to heterogeneous bonds and to enhance the stability of ChG film [23]. However, being in the temperature of could also cause cracks in the film during the waveguide fabrication process [21] [also shown in Fig. 2(d)]. As a consequence, a relatively moderate temperature (much lower than ) is always chosen to obtain a compromised annealing effect [24]. We had previously proposed a light-annealing process and demonstrated that, when an as-deposited film was subjected to illumination with bandgap light by an array of external halogen lamps, its RI is effectively pushed toward that of the bulk glass, which solved the cracking issue during the thermal annealing [21]. However, the reported film quality remains low compared with that by conventional thermal annealing processes. A possible reason is that, during the transfer process, the film will also be introduced with additional impurity due to the fact that the thermal annealing oven and the thermal evaporation equipment are separated. Up to date, the factors of ChG MRs, such as microspheres and microdisks, have been improved up to and , respectively [25,26]. However, the highly delocalized optical modes in these ChG MRs render them challenging for compact nonlinear optical parametric applications requiring dispersion engineering [27].
Generally, the OPO generation in MRs depends on three aspects: 1) the nonlinear figure of merit [FOM, , where is the Kerr nonlinearity, and is the TPA coefficient] of the platform [8]; 2) the high -factor and strong modal confinement as well as the large spatial overlap between modes, which are essential for gain to overcome cavity loss [28]; 3) the GVD engineering of the waveguide can achieve simultaneous frequency- and phase-matching among the pump, signal, and idler modes [29]. Over the past years, researches on chalcogenide photonics have focused on the development of new ChG material components with ultrahigh nonlinearity. Yet, it remains challenging to implement high-quality ChG films for the fabrication of integrated nonlinear devices. Thus far, there are few reports on solving chalcogenide films’ stability and realizing the OPO process in ChG MRs [22].
Sign up for Photonics Research TOC. Get the latest issue of Photonics Research delivered right to you!Sign up now
To address these issues, as shown in Fig. 1, we introduce an
Figure 1.(a) Schematic of the improved
2. EXPERIMENT
A. Preparation of High Purity ChG Glasses
High purity elements (99.9999% As and 99.9999% S) were used to prepare the glass by the conventional melt-quenching technique. The high purity glass was fabricated by a modified physical and chemical purification technique [31]. The purified glass was used as the starting glass for ChG film by thermal deposition.
B. Preparation and Annealing Post-treatment of ChG Film
The films were deposited on Si substrates with a 3 μm layer by thermal evaporation in a vacuum chamber at a base pressure of [32]. First, the substrate was mounted on a rotation holder and pretreated using Ar plasma to improve the adhesion between the films and substrates. The evaporation rate was set to approximately 5–6 nm/min. Note, the temperature of the substrate was maintained at 30°C during the film deposition process.
As shown in Fig. 1(a), a power-tunable laser panel with a center wavelength of 532 nm was installed on a screw that could rotate in the deposition chamber. After the film deposition, the laser panel was raised to 5 cm below the film by rotating 90°. Moreover, the ChG wafer loaded on the top of the chamber was rotated with a speed of 20 revolutions/min, and thereby the power densities of the laser panel could be uniform over a full 4 in. diameter wafer. After that, the vacuum was broken, and the wafer was transferred into an atomic layer deposition (ALD) chamber. A passivation layer () with a thickness of 2 nm was deposited on the surface of the chip by ALD, which is to protect the waveguide from moisture. For comparison, a set of 850 nm thick films was put into a vacuum furnace and annealed at different temperatures (e.g., 130°C, 150°C, and 180°C) for 24 h to ensure molecular bond conversion finishes in the films [21]. After that, the temperature dropped in the furnace with a cooling rate of around 5°C/min. The ChG films were cut into for both the light-induced annealing and thermal annealing studies.
3. RESULTS
A. Improvement of Stability of ChG Film
Figure 2.(a) Composition of
The Raman and RI spectra of the films by light annealing under different laser intensities of light and duration were measured to achieve optimized parameters of the light-annealing process. As shown in Figs. 3(a)–3(d), the rate of homopolar bond conversions increases as the increasement of power densities of light and the Raman spectra of the three films are gradually approaching a uniform and stable state after 900 min of exposure to the laser. Compared with T180, the light-annealing process can make the film’s RI much closer to bulk glass. Moreover, the FILM-L’s RI is closer to that of bulk glass as the laser power increased. In particular, when the laser intensity is increased to , the film’s RI is the same as that of glass, which indicates that higher laser intensity can make the crosslinking of the film molecule close to the bulk glass (Fig. 2). Compared with full thermal annealing process (about 24 h), the light-induced annealing process significantly reduces the annealing time (less than 900 min). As shown in Fig. 4, we measured these films’ roughness, including FILM-L, FILM-D, and FILM-T, through atomic force microscopy (AFM) [27]. The roughness of the T180 () is significantly higher than that of FILM-D (). However, the roughness of films annealed under different laser intensity conditions ( and 0.680 nm corresponding to 10 and , respectively) is slightly higher than that of FILM-D. Finally, we chose and 900 min as the light-annealing power density and duration, respectively. To avoid film contamination or degradation during the transfer of the film from the vacuum chamber to the annealing equipment, an
Figure 3.Raman Spectra of
Figure 4.3D AFM scan images of the surface of ChG films: by light annealing under power densities of (a)
B. Reduction of Propagation Loss of ChG Waveguides
Low-loss on-chip ChG photonic devices are critical for nonlinear photonic applications. Typically, the loss sources of planar waveguides are from the surface interactions and material absorptions [27]. For thermally evaporated ChG films, the material absorptions mainly come from the air’s impurity and oxidized homogeneous bonds. According to the optical microscope images shown in Figs. 1(d) and 1(e), it can be found that there are many bright spots on the surface of FILM-D, which are gradually formed in the air, while FILM-L is highly stable and has not been oxidized. To obtain smooth waveguide sidewalls, we optimize the waveguide fabrication process during etching and post-processing to reduce roughness. Typically, standard waveguide fabrication methods for a ChG waveguide include patterning a masking layer using electron-beam lithography (EBL) or photo-lithography (PL) and transferring this pattern into the waveguide device layer using reactive ion etching (RIE) with fluorine etching gas. Trifluoromethane () gas and oxygen () plasma treatment are widely used as etchants in ChG fabrication, and residual resists are removed by inductively coupled plasma reactive ion etching (ICP-RIE) [33]. The roughness of the E-beam resists edge will be transferred to the sidewall, and the fluorocarbon polymer will also be formed and attached to the sidewall during the process. In this paper, a modified waveguide fabrication process, including multipass optimized plasma etching conditions and the waveguide’s thermal reflow process, is shown in Fig. 5(a).
Figure 5.(a) Fabrication process for
Moreover, we investigated the chamber pressure, etching gas flow rate (), radio-frequency (RF) power, and Ar gas flow rate to determine the optimal etching recipe, as shown in Table 2. Herein, the etching rate of ChG film is 100 nm/min, and the etching selection ratio of ARP6200/ChG configuration is 1:5. The waveguides with a cross-sectional dimension of are fabricated. As shown in Fig. 5(b), after removing the passivation layer on the sidewall surface by the second plasma treatment, the waveguide sidewall is very smooth. For comparison, the insertion losses of ChG waveguides with different lengths of 8, 26.7, 45.4, 64.1, and 82.8 mm were measured using the cut-back method. The microscope image of the ChG spiral waveguide with a length of 82.8 mm is shown in Fig. 5(c). After removing the residual resist, an 82.8 mm spiral waveguide was fabricated with a loss as low as 0.22 dB/cm [as line b in Fig. 5(d)]. Moreover, to verify the effect of thermal reflow, the propagation loss of the BCB-cladding waveguide has been effectively reduced by a thermal reflow process [line c in Fig. 5(d)]. As shown in Fig. 5(e), compared with the loss of waveguides reflowed at 120°C, 160°C, and 180°C, respectively, we can observe the lowest loss of the waveguide by thermal reflowing at 140°C. The loss difference mainly comes from the changes in the roughness of the waveguide surface [27]. The reflow process at low temperature () will reduce the roughness of the waveguide surface, while high-temperature treatment () will increase surface scattering loss due to internal stress [21]. Therefore, the thermal reflow temperature of the waveguide was set to 140°C. BCB polymer is not the critical factor in reducing propagation loss. We tried other polymer claddings, such as SU-8 2000 and IPG, and achieved similar results. The equal shrinkage coefficient of the ChG core and polymer cladding and moderate thermal treatment can make the waveguide sidewall’s surface smoother. This is a unique method to change the appearance of the ChG device surface [34]. Summary of the RIE and ICP-RIE Conditions RIE is used to fabricate waveguides; ICP-RIE is used to remove resist. RIE ICP Chemistry Pressure (mTorr) 10 10 RF power (W) 100 10 ICP power (W) 300
C. Design and Fabrication of High-
Figure 6.(a) Simulated dispersion of
Table 3 shows the comparison of different ChG platforms for nonlinear photonics. Here, we use the attenuation-related defined by [35] and TPA-related figure of merit (, defined by , is the TPA coefficient). We should note that fabricating low-loss waveguides and microrings has been demonstrated for several ChGs. At the same time, our works now offer both high and among dispersion-engineered ChGs devices, and especially the highest value of has been achieved.
D. OPO in the High
Here, we experimentally demonstrate an OPO process in the improved ChG microring. An experimental setup is shown in Fig. 7(a). A continuous-wave (CW) as pump light was generated through a tunable laser (Toptica CTL 1550). The pump light was amplified by an erbium-doped fiber amplifier and subsequently attenuated by a variable optical attenuator to control the input power. The polarization of pump light was aligned with polarization controllers (PCs) to TM polarization of the ChG waveguide. Finally, the output was detected by a photoelectric detector (PD), and the OPO process was shown in an oscilloscope. For OPO measurement, two inverse tapers were fabricated for lensed fiber-chip-lensed fiber couplers to achieve the fiber-to-chip coupling loss of 3.5 dB per facet. The result of the OPO spectrum was recorded by an optical spectrum analyzer. Figure 7(b) shows the OPO spectrum of such a 183 GHz resonator operated at 7 mW, where primary sidebands at spaced from pump mode can be observed. To determine the threshold power of the OPO process, we measured the output power of the first generated sideband for different pump powers in Fig. 7(c). The threshold power is around 5.41 mW, which is comparable with the calculated threshold power of 5.03 mW by considering this expression [29]:
Figure 7.(a) Schematic of the OPO measurement setup. (b) Measured OPO spectrum for input power of 7 mW (
5. CONCLUSION
An
References
[1] X. Liu, C. Sun, B. Xiong, L. Wang, J. Wang, Y. Han, Z. Hao, H. Li, Y. Luo, J. Yan, T. Wei, Y. Zhang, J. Wang. Integrated high-
[2] J. Liu, A. S. Raja, M. Karpov, B. Ghadiani, M. H. P. Pfeiffer, B. Du, N. J. Engelsen, H. Guo, M. Zervas, T. J. Kippenberg. Ultralow-power chip-based soliton microcombs for photonic integration. Optica, 5, 1347-1353(2018).
[3] M. Kues, C. Reimer, J. M. Lukens, W. J. Munro, A. M. Weiner, D. J. Moss, R. Morandotti. Quantum optical microcombs. Nat. Photonics, 13, 170-179(2019).
[4] A. V. Muraviev, V. O. Smolski, Z. E. Loparo, K. L. Vodopyanov. Massively parallel sensing of trace molecules and their isotopologues with broadband subharmonic mid-infrared frequency combs. Nat. Photonics, 12, 209-214(2018).
[5] D. K. Armani, T. J. Kippenberg, S. M. Spillane, K. J. Vahala. Ultra-high-
[6] W. Chen, S. K. Ozdemir, G. Zhao, J. Wiersig, L. Yang. Exceptional points enhance sensing in an optical microcavity. Nature, 548, 192-196(2017).
[7] X. Shen, R. C. Beltran, V. M. Diep, S. Soltani, A. M. Armani. Low-threshold parametric oscillation in organically modified microcavities. Sci. Adv., 4, eaao4507(2018).
[8] B. J. Eggleton, B. Luther-Davies, K. Richardson. Chalcogenide photonics. Nat. Photonics, 5, 141-148(2011).
[9] H. T. Lin, Y. Song, Y. Z. Huang, D. Kita, S. Deckoff-Jones, K. Q. Wang, L. Li, J. Y. Li, H. Y. Zheng, Z. Q. Luo, H. Z. Wang, S. Novak, A. Yadav, C. C. Huang, R. J. Shiue, D. Englund, T. Gu, D. Hewak, K. Richardson, J. Kong, J. J. Hu. Chalcogenide glass-on-graphene photonics. Nat. Photonics, 11, 798-805(2017).
[10] C. R. Petersen, I. Møller, , B. Zhou, S. Dupont, J. Ramsay, T. Benson, S. Sujecki, N. Abdel-Moneim, Z. Tang, D. Furniss, A. Seddon, O. Bang. Mid-infrared supercontinuum covering the 1.4–13.3 μm molecular fingerprint region using ultra-high NA chalcogenide step-index fibre. Nat. Photonics, 8, 830-834(2014).
[11] L. Li, H. Lin, S. Qiao, Y. Zou, S. Danto, K. Richardson, J. D. Musgraves, N. Lu, J. Hu. Integrated flexible chalcogenide glass photonic devices. Nat. Photonics, 8, 643-649(2014).
[12] D.-G. Kim, S. Han, J. Hwang, I. H. Do, D. Jeong, J.-H. Lim, Y.-H. Lee, M. Choi, Y.-H. Lee, D.-Y. Choi, H. Lee. Universal light-guiding geometry for on-chip resonators having extremely high
[13] B. Morrison, A. Casas-Bedoya, G. Ren, K. Vu, Y. Liu, A. Zarifi, T. G. Nguyen, D.-Y. Choi, D. Marpaung, S. J. Madden, A. Mitchell, B. J. Eggleton. Compact Brillouin devices through hybrid integration on silicon. Optica, 4, 847-854(2017).
[14] H. G. Winful, I. V. Kabakova, B. J. Eggleton. Model for distributed feedback Brillouin lasers. Opt. Express, 21, 16191-16199(2013).
[15] T. F. Büttner, I. V. Kabakova, D. D. Hudson, R. Pant, C. G. Poulton, A. C. Judge, B. J. Eggleton. Phase-locking and pulse generation in multi-frequency Brillouin oscillator via four wave mixing. Sci. Rep., 4, 5032(2014).
[16] S. Xing, D. Grassani, S. Kharitonov, L. Brilland, C. Caillaud, J. Trolès, C.-S. Brès. Mid-infrared continuous-wave parametric amplification in chalcogenide microstructured fibers. Optica, 4, 643-648(2017).
[17] M. Bernier, V. Fortin, M. El-Amraoui, Y. Messaddeq, R. Vallee. 3.77 µm fiber laser based on cascaded Raman gain in a chalcogenide glass fiber. Opt. Lett., 39, 2052-2055(2014).
[18] T. J. Kippenberg, R. Holzwarth, S. A. Diddams. Microresonator-based optical frequency combs. Science, 332, 555-559(2011).
[19] T. J. Kippenberg, A. L. Gaeta, M. Lipson, M. L. Gorodetsky. Dissipative Kerr solitons in optical microresonators. Science, 361, eaan8083(2018).
[20] D. H. Broaddus, M. A. Foster, I. H. Agha, J. T. Robinson, M. Lipson, A. L. Gaeta. Silicon-waveguide-coupled high-
[21] D.-Y. Choi, A. Wade, S. Madden, R. Wang, D. Bulla, B. Luther-Davies. Photo-induced and thermal annealing of chalcogenide films for waveguide fabrication. Phys. Procedia, 48, 196-205(2013).
[22] A. L. Gaeta, M. Lipson, T. J. Kippenberg. Photonic-chip-based frequency combs. Nat. Photonics, 13, 158-169(2019).
[23] A. El-Sebaii, S. A. Khan, F. Al-Marzouki, A. Faidah, A. Al-Ghamdi. Role of heat treatment on structural and optical properties of thermally evaporated Ga10Se81Pb9 chalcogenide thin films. J. Lumin., 132, 2082-2087(2012).
[24] B. J. Kim, J. H. Kim, S. H. Hwang, A. S. Budiman, H. Y. Son, K. Y. Byun, N. Tamura, M. Kunz, D. I. Kim, Y. C. Joo. Microstructure evolution and defect formation in Cu through-silicon vias (TSVs) during thermal annealing. J. Electron. Mater., 41, 712-719(2012).
[25] J. Zhu, M. Zohrabi, K. Bae, T. M. Horning, M. B. Grayson, W. Park, J. T. Gopinath. Nonlinear characterization of silica and chalcogenide microresonators. Optica, 6, 716-722(2019).
[26] Q. Du, Y. Huang, J. Li, D. Kita, J. Michon, H. Lin, L. Li, S. Novak, K. Richardson, W. Zhang, J. Hu. Low-loss photonic device in Ge–Sb–S chalcogenide glass. Opt. Lett., 41, 3090-3093(2016).
[27] X. Ji, F. A. S. Barbosa, S. P. Roberts, A. Dutt, J. Cardenas, Y. Okawachi, A. Bryant, A. L. Gaeta, M. Lipson. Ultra-low-loss on-chip resonators with sub-milliwatt parametric oscillation threshold. Optica, 4, 619-624(2017).
[28] M. Pu, L. Ottaviano, E. Semenova, K. Yvind. Efficient frequency comb generation in AlGaAs-on-insulator. Optica, 3, 823-826(2016).
[29] T. J. Kippenberg, S. M. Spillane, K. J. Vahala. Kerr-nonlinearity optical parametric oscillation in an ultrahigh-
[30] M. R. Lamont, B. Luther-Davies, D. Y. Choi, S. Madden, B. Eggleton. Supercontinuum generation in dispersion engineered highly nonlinear (γ=10 /W/m) As2S3 chalcogenide planar waveguide. Opt. Express, 16, 14938-14944(2008).
[31] B. Zhang, W. Guo, Y. Yu, C. Zhai, S. Qi, A. Yang, L. Li, Z. Yang, R. Wang, D. Tang, G. Tao, B. Luther-Davies, P. Lucas. Low loss, high NA chalcogenide glass fibers for broadband mid-infrared supercontinuum generation. J. Am. Ceram. Soc., 98, 1389-1392(2015).
[32] J. Hu, M. Torregiani, F. Morichetti, N. Carlie, A. Agarwal, K. Richardson, L. C. Kimerling, A. Melloni. Resonant cavity-enhanced photosensitivity in As2S3 chalcogenide glass at 1550 nm telecommunication wavelength. Opt. Lett., 35, 874-876(2010).
[33] Y. Zhu, L. Wan, Z. Chen, Z. Yang, D. Xia, P. Zeng, J. Song, J. Pan, Y. Feng, M. Zhang. Effects of shallow suspension in low-loss waveguide-integrated chalcogenide microdisk resonators. J. Lightwave Technol., 38, 4817-4823(2020).
[34] J. J. Kaufman, G. Tao, S. Shabahang, E.-H. Banaei, D. S. Deng, X. Liang, S. G. Johnson, Y. Fink, A. F. Abouraddy. Structured spheres generated by an in-fibre fluid instability. Nature, 487, 463-467(2012).
[35] M. E. Marhic, P. A. Andrekson, P. Petropoulos, S. Radic, C. Peucheret, M. Jazayerifar. Fiber optical parametric amplifiers in optical communication systems. Laser Photon. Rev., 9, 50-74(2015).
[36] S. Madden, D.-Y. Choi, D. Bulla, A. V. Rode, B. Luther-Davies, V. G. Ta’eed, M. Pelusi, B. Eggleton. Long, low loss etched As2S3 chalcogenide waveguides for all-optical signal regeneration. Opt. Express, 15, 14414-14421(2007).
[37] M. J. Collins, A. S. Clark, J. He, D.-Y. Choi, R. J. Williams, A. C. Judge, S. J. Madden, M. J. Withford, M. Steel, B. Luther-Davies, C. L. Xiong, B. J. Eggleton. Low Raman-noise correlated photon-pair generation in a dispersion-engineered chalcogenide As2S3 planar waveguide. Opt. Lett., 37, 3393-3395(2012).
[38] X. Gai, S. Madden, D. Y. Choi, D. Bulla, B. Luther-Davies. Dispersion engineered Ge11.5As24Se64.5 nanowires with a nonlinear parameter of 136W−1m−1 at 1550nm. Opt. Express, 18, 18866-18874(2010).
[39] Q. Du, Z. Luo, H. Zhong, Y. Zhang, Y. Huang, T. Du, W. Zhang, T. Gu, J. Hu. Chip-scale broadband spectroscopic chemical sensing using an integrated supercontinuum source in a chalcogenide glass waveguide. Photon. Res., 6, 506-510(2018).
[40] B. Zhang, Y. Yu, C. Zhai, S. Qi, Y. Wang, A. Yang, X. Gai, R. Wang, Z. Yang, B. Luther-Davies, Y. Xu. High brightness 2.2–12 μm mid-infrared supercontinuum generation in a nontoxic chalcogenide step-index fiber. J. Am. Ceram. Soc., 99, 2565-2568(2016).
[41] Y. Yu, B. Zhang, X. Gai, C. Zhai, S. Qi, W. Guo, Z. Yang, R. Wang, D. Y. Choi, S. Madden, B. Luther-Davies. 1.8-10 µm mid-infrared supercontinuum generated in a step-index chalcogenide fiber using low peak pump power. Opt. Lett., 40, 1081-1084(2015).
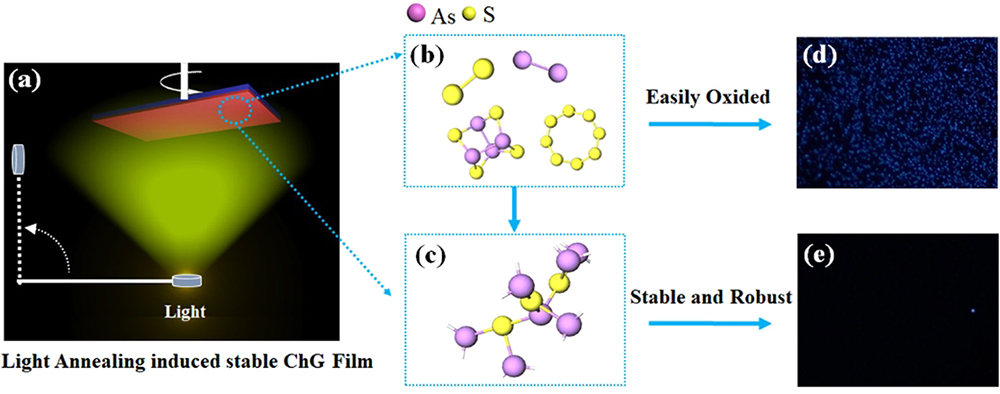
Set citation alerts for the article
Please enter your email address