Abstract
The concept of using plasmonic nanostructures to manage light in solar cells has offered an unprecedented potential for dramatically increased solar energy conversion efficiency that breaks the previously predicated efficiency limit. In the past decade, intensive research efforts have been focused on this field. However, nanoplasmonic solar cells still remained in the laboratory level. To facilitate the transformation of the nanoplasmonic solar cell concept to a viable high-efficiency technology solution for the solar industry, it is essential to address key fundamental as well as practical challenges including the detrimental absorption of metallic nanostructures, narrow-band absorption enhancement in the active layer, the high cost and scarcity of noble metals, and the expensive and complicated plasmonic nanomaterial fabrication and integration methods. In this paper, after a brief review of our main results in nanoplasmonic solar cells, we present our strategies for using innovative photonic methods to overcome these challenges and demonstrate a large-area (173 cm2) broadband plasmonic thin-film solar minimodule with an efficiency of 9.5% resulting from the enhanced plasmonic light scattering enabled by silver lumpy nanoparticles with an ultralow nanoparticle coverage density of 5%.1. INTRODUCTION
The past 10 years have witnessed explosive efforts in plasmonic solar cell research, which has led to the exploration of both new science and new solar cell architectures toward enhanced solar cell performance, which is challenging to achieve through conventional semiconductor engineering methods [1–20]. Among various proposed plasmonic structures, nanoparticles are highly preferable because of their strong scattering properties, tunable by particle size, shape, and dielectric environment, and also because of their simple preparation and integration methods that can be readily compatible with the standard solar cell fabrication process without dramatically increasing the cost [7–20]. Metallic nanoparticles have been demonstrated to be effective in improving the short circuit current [7–20], the fill factor [15,16,19], and the energy conversion efficiency [7,8,15,16,19] of solar cells. In particular, it has been shown that through implementing tailored metallic nanoparticles, ultrathin amorphous silicon (a-Si) solar cells can be achieved [16], providing promising solutions for high-efficiency and low-cost solar cells.
In spite of the great success, all of the current plasmonic solar cell research has been limited to the proof-of-concept level in the laboratory, which means that only small-sized solar cells of a few millimeters to a couple of centimeters have been investigated. Therefore, this research is far away from practical application. To scale up the technology effective for the industry uptake, the key outstanding fundamental as well as practical challenges have to be solved. These include designing an innovative nanoparticle architecture to minimize the detrimental absorption loss and in the meantime enable broadband and strong light scattering that could significantly reduce the usage (the coverage density) of metal nanoparticles, identifying new low-cost and earth abundant materials to function as the plasmonic nanomaterials, and developing an effective and inexpensive integration method that can ensure a large-range uniform distribution of nanoparticles to achieve the maximum solar cell performance. In this paper, we present our strategies to systematically tackle these bottle-neck issues. We designed and fabricated the “lumpy” nanoparticles to minimize the detrimental nanoparticle absorption and in the meantime to maximize the beneficial broadband scattering [9,21,22], we identified and demonstrated that Al nanoparticles have superior light-trapping capability outperforming conventional plasmonic materials, Ag and Au [11,22], and we developed innovative drop-casting, dip-coating, and electroless deposition methods for uniform and large-scale integration of metallic nanoparticles with silicon solar cells [10,15,18].
To be more specific, in the last section of this paper we demonstrate an upscaled thin-film silicon solar minimodule consisting of 13 solar cells with a total area of by integrating the strongly scattering broadband lumpy nanoparticles [9,21,22] through a multistep drop-casting method. The short circuit current density () enhancement mapping produced from the external quantum efficiency (EQE) measurement confirms the uniformity of the nanoparticle distribution over the entire solar minimodule. Due to the superior scattering properties of the lumpy nanoparticles and their large-range uniform distribution, the coverage density was able to be minimized to achieve optimized enhancement. More than 12% efficiency enhancement was achieved at a coverage density of only 5% due to the dominant increases in and the fill factor. In addition we have also developed an effective procedure to ensure the nanoparticles can be compatible with the multistep laser scribing process without having a detrimental effect on solar cell performance.
Sign up for Photonics Research TOC. Get the latest issue of Photonics Research delivered right to you!Sign up now
2. STRATEGIES FOR CREATING UPSCALED PLASMONIC SOLAR CELLS
A. Lumpy Nanoparticle-enabled Broadband Solar Cell Absorption Enhancement
Plasmonic resonant scattering is one of the major and effective mechanisms in most of the metallic nanoparticle-enabled solar cells [1,5]. Enhanced scattering from metallic nanoparticles caused by plasmonic resonances is normally restricted to certain resonant wavelengths determined by the size, shape, and local dielectric environment of the nanoparticles [1,5]. In addition, these enhancements are generally offset by the detrimental particle absorption at other wavelengths, therefore hardly leading to any substantial efficiency boost of the solar cells. To make full use of the solar spectrum, broadband absorption enhancement is the primary challenge to tackle before the plasmonic solar cells can take off from laboratories. To achieve broadband absorption enhancement in the silicon layer, the plasmonic nanostructures need to be designed to strongly scatter the incident broadband solar light into a large angle range so that light can be best trapped inside the silicon layer while keeping the particle absorption at a minimum.
Based on the scattering characteristics of small and large nanoparticles, we designed and demonstrated a “lumpy” nanoparticle geometry. Instead of having a smooth surface like conventional nanoparticles, the lumpy nanoparticle consists of a large silver core particle surrounded by small particles to effectively scatter light in a broad spectrum range with large oblique angles and in the meantime minimize the detrimental particle absorption [9,21,22]. Such lumpy Ag nanoparticles were synthesized by the simple and low-cost wet-chemical method and integrated at the back of silicon thin-film solar cells. Significant enhancement in and the fill factor was achieved, as shown in Fig. 1, as a result of the effective scattering from the lumpy nanoparticles and the reduced contact resistivity due to the Ag nanoparticles, leading to a dramatically boosted efficiency of 23%.
![Jsc enhancements of thin-film a-Si solar cells integrated with Ag lumpy nanoparticles and smoothly surfaced nanoparticles with the same size and size distribution. Inset: schematic drawings of the integration of the lumpy Ag particle with solar cells. [Reproduced with permission from Ref. 21. Copyright The Optical Society 2013.]](/richHtml/prj/2013/1/1/01000022/img_001.jpg)
Figure 1. enhancements of thin-film a-Si solar cells integrated with Ag lumpy nanoparticles and smoothly surfaced nanoparticles with the same size and size distribution. Inset: schematic drawings of the integration of the lumpy Ag particle with solar cells. [Reproduced with permission from Ref. 21. Copyright The Optical Society 2013.]
B. High-Performance and Low-Cost Al Nanoparticles
Noble metals (Ag and Au) have been the most widely used nanomaterials in the laboratory due to their surface plasmon resonances located in the visible range, and therefore they interact more strongly with the peak solar intensity [1–10,12–21]. However, such noble metal nanoparticles on the front surface of solar cells always introduce reduced light absorption in silicon at short wavelengths due to the Fano effect [23], i.e., the destructive interference between scattered and unscattered light that occurs below the resonance. In addition, noble metal nanoparticles are impractical for large-scale solar cell manufacture due to their high cost and scarcity in the earth crest.
Recently, we systematically investigated the light-trapping behavior of the low-cost and earth abundant Al nanoparticles on the front surface of silicon wafer solar cells and compared it with light trapping of Ag and Au nanoparticles using the finite-difference time-domain method [10,21,23,24]. Encouragingly, we found that the photon absorption of Al nanoparticle-integrated silicon solar cells is much higher than that of Ag and Au nanoparticle-integrated cells, as shown in Fig. 2, which is due to (a) the surface plasmon resonance of Al lying in the ultraviolet range, which potentially leads to a much less negative impact to the solar cell performance caused by the destructive interference effect, and (b) a broadband light absorption enhancement matching well with the solar spectrum. More interestingly we found that Al nanoparticles in combination with the conventional SiNx antireflection coating can lead to a 4.3% enhancement in the light absorption in silicon compared with a standard SiNx.
In spite of the obvious advantages predicated by theory, Al nanoparticle-enhanced solar cells have not yet been realized due to the great challenges associated with the synthesis and control of the low surface coverage simultaneously. An Al nanostructure is too active to be produced by the wet-chemical method at ambient temperature. Although it can be obtained by evaporating a metal thin film onto the solar cell surface followed by an annealing process, it is difficult to control the surface coverage below 30% [25]. Under such a high coverage, the nanoparticles block a significant amount of light, leading to reduced solar cell absorption. Recently we developed an innovative physical method based on modified evaporation [26] to prepare Al nanoparticle suspensions. We also integrated the Al nanoparticles on the front side of textured screen-printed solar cells with a nanoparticle coverage density as low as 10% [26,27]. A broadband solar cell absorption enhancement was confirmed by the EQE measurement, leading to 6.3% improvement in and 6.1% improvement in the energy conversion efficiency. This work provides a low-cost and high-efficiency solution for practical large-scale implementation of plasmonic nanoparticles for solar cell performance enhancement.
Sign up for Photonics Research TOC. Get the latest issue of Photonics Research delivered right to you!Sign up now
C. Effective and Inexpensive Integration Method
Once high-performance and low-cost metallic nanoparticles are available, an effective, simple, and inexpensive integration method becomes critical for full-scale nanoplasmonic solar cells. It is highly preferred that the integration method is capable of upscale and can be readily compatible with the standard solar cell fabrication process without significantly increasing the cost. To this end, we have developed the drop-casting, dip-coating, and electroless deposition methods to integrate nanoparticles with solar cells. All of these methods are industry-friendly and capable of full-scale solar cell process with fine control of the nanoparticle surface coverage and large-scale uniformity. In this paper, we give an example of the dip-coating method [10].
As shown in the schematic drawing of the dip-coating process in Fig. 3, before dipping, solar cells need to be washed with ethanol and dried by gas. A solution containing size and concentration tailored nanoparticles is prepared first. To integrate the nanoparticles with the solar cells, the solar cells need to be mounted on the sample holder of the dip coater (KSV company, model: DS), vertically dipped into the colloidal nanoparticle solution, immersed for 4 min, and pulled out of the solution with an optimized velocity; then they are left clamped on the sample holder until being dried in air at room temperature. Optimizing the dipping conditions, including the pulling speed, immersion time, and solution concentration, allows control of the particle density. It was found that reducing the pulling speed increases the surface coverage. A higher particle coverage of about 80% on silicon substrate could also be achieved through a multiple-step dip coating. Therefore, the dip-coating method is fast, controllable, and easy to use in the integration of nanoparticles into solar cells. Moreover, one can form the particle layer with a high throughput without wasting particles, compared to other coating methods such as spin coating, which suffers from difficulties in delivering the exact amount of nanoparticles onto the substrate, as well as difficulties in delivering particles onto a nonplanar substrate.
![Spectral characteristics of Si absorbance for the optimized Al (green), Ag (blue), and Au (red) nanoparticles placed on the front surface of an Si wafer compared with bare silicon. [Reproduced with permission from [11]. Copyright The American Institute of Physics 2012.]](/Images/icon/loading.gif)
Figure 2.Spectral characteristics of Si absorbance for the optimized Al (green), Ag (blue), and Au (red) nanoparticles placed on the front surface of an Si wafer compared with bare silicon. [Reproduced with permission from [11]. Copyright The American Institute of Physics 2012.]
![Schematic diagram depicting the dip-coating process to integrate nanoparticles (NPs onto the top surface of silicon solar cells (Scs). The real photograph of the experimental setup is included. [Reproduced with permission from [10]. Copyright The Optical Society 2012.]](/Images/icon/loading.gif)
Figure 3.Schematic diagram depicting the dip-coating process to integrate nanoparticles (NPs onto the top surface of silicon solar cells (Scs). The real photograph of the experimental setup is included. [Reproduced with permission from [10]. Copyright The Optical Society 2012.]
3. UPSCALED BROADBAND PLASMONIC THIN-FILM SOLAR MODULES
The availability of low-cost, high-performance nanoparticles and simple and inexpensive integration methods allows us to develop upscaled broadband plasmonic solar modules of value to the solar industry. In this section, we demonstrate in detail a comparably large-scale high-performance solar module developed using the nanoplasmonic concept as an example.
A. Fabrication of Plasmonic Thin-Film Solar Modules
The schematic diagram of the solar minimodule used in this work is shown in Fig. 4(a). It consists of 13 a-Si thin-film solar cells connected to each other in series. Each cell has an effective area of . The cells had a geometry of glass/transparent conductive oxide (TCO)/p-i-n a-Si/ZnO/Ag, as shown in the magnified cross-sectional diagram in Fig. 4(b). Before depositing the silicon layer, the TCO glass was scribed by a UV laser to form 13 isolated regions as the positive electrode. The silicon layers (300 nm in total) were then deposited by plasma-enhanced chemical vapor deposition at an RF excitation frequency. A green laser beam was used afterward to scribe the silicon layer to form 13 cells, which were slightly offset to the first round laser scribing, as shown in Fig. 4(b). Silver lumpy nanoparticles of 200 nm in diameter were selected according to our previous study [9] and embedded inside the ZnO:Al layer 20 nm away from the silicon layer at the rear side of the solar cells. After integration of the nanoparticles, another ZnO:Al layer was deposited by the RF sputtering on top of the nanoparticles. The silver back contact layer was fabricated finally by the RF sputtering. In the end the third round laser scribing of the entire ZnO:Al layer was conducted. Special caution needs to be given to the laser scribing process, as it might cause the metal nanoparticles remaining in the scribed channels to be in contact with the silicon layer, which leads to shunting of the solar cells. A thorough ultrasound cleaning after each laser scribing process seemed to be effective to remove all of the nanoparticle residuals in the scribed channels.
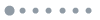
Figure 4.(a) Schematic diagram showing the structure of the plasmonic solar module. (b) Enlarged schematic cross section of the solar cells integrated with silver lumpy nanoparticles. (c) Photo of the plasmonic thin-film module. The white circles provide an example of where the EQE is measured on a solar cell for the mapping.
B. Photovoltaic Application of Silver Lumpy Particles in Solar Minimodules
It was found in our experiment that for the fixed-sized nanoparticles, the solar cell performance was highly sensitive to the nanoparticle coverage density and their distribution uniformity on the solar cells. A nonuniform particle distribution produces agglomeration, leading to a reduced scattering strength and a deteriorated electrical property due to the poor contact, which eventually degrades the solar cell efficiency. This becomes a serious problem when building a plasmonic thin-film solar module, because if the efficiencies of individual cells deviate too much from each other, the output of the entire module can be downgraded. On the other hand, a uniform distribution can make full use of the scattering cross-sectional area of each particle, maximizing the area that a single particle can cover on the solar cells, therefore significantly reducing the number of nanoparticles required (i.e., the coverage density).
To ensure a large-range uniform distribution of the silver nanoparticles, a multistep drop-casting integration method was developed. In each step of drop casting, nanoparticles with a low coverage density of 1.25% was evenly spread on the ZnO:Al layer followed by a low temperature heating at 50°C to quickly evaporate the solution and dry the nanoparticles uniformly on the surface. To reach high coverage density, this step was repeated. Different coverage densities of 2.5%, 5%, 10%, and 12.5% were investigated. Interestingly we found a low coverage density of 5% provides the best solar cell performance enhancement, which was only half the coverage density we used for the small area () solar cells [9]. We attribute this to the highly uniform distribution of the nanoparticles, which leads to the more efficient usage of all the particles.
The uniform light-trapping effect from the nanoparticles was examined by mapping the short circuit current enhancement from the EQE measurement (PV Measurement QEX7) over the entire area of the solar minimodule with a 5% coverage density compared with a reference module without nanoparticles, as shown in Fig. 5. The mapping was conducted by measuring the EQE of each individual cell at five different positions, as indicated from the examples given in Fig. 4(c) (marked as the white circles). The measured enhancement shows a high uniformity over the entire module area with a minimum value of 1.04 and a maximum value 1.07, which leads to a variation of only 2.5%. Since the normalized enhancement is only correlated to the light-trapping effect induced by the nanoparticles, this result proves that the distribution of nanoparticles across the entire module is uniform. The positive enhancement indicates that the nanoparticles are effective in improving the light trapping in the thin-film solar module.
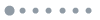
Figure 5. enhancement mapping produced from the EQE data for the solar minimodule containing 13 small solar cells integrated with 200 nm lumpy nanoparticles at a 5% coverage density compared with the reference module without nanoparticle integration.
It is well known that even for a thin-film solar module without nanoparticles, the value at different positions of the solar cells is varying in a small range due to the slight difference in the film properties. This problem becomes worse for large-scale solar modules, which poses a challenge in identifying the exact impact from the nanoparticles if the performance of individual cells before and after integration of nanoparticles is compared. To rule out the influence from individual cell differences, we analyzed the statistics of the , the open circuit voltage (), the fill factor, and the efficiency of all the cells of the minimodule before and after the nanoparticle integration using the current-voltage () measurement under a simulated AM1.5 spectrum (Oriel-Sol 3A and Keithley 2400 source meter). The results are shown in Fig. 6.
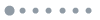
Figure 6.Statistics of the major parameters: (a) , (b) fill factor, (c) , and (d) efficiency of the solar cells in the solar modules with (red) and without (blue) the nanoparticle integration.
It can be seen from Fig. 6(a) that compared with the solar cells in the reference module, the solar cells integrated with nanoparticles present an obvious increase in due to the strong plasmonic scattering from the lumpy nanoparticles, which helps to trap more light into the solar cells [9,21]. The value of the majority of cells is improved from 14.6 to , leading to an enhancement of approximately 5.5%, which is much larger than that observed in the previous study with the same surface coverage [9]. We attribute this reduced optimized surface coverage to the effective usage of each nanoparticle because of the highly uniform distribution of nanoparticles. Due to the reduced contact resistivity of the dielectric layer by incorporating the silver nanoparticles, the peak of the fill factor distribution is shifted from 0.63 to 0.66, increased by 4.7%, as shown in Fig. 6(b). In contrast, the peak of the distribution shifts only slightly from 0.87 to 0.88 V [Fig. 6(c)]. As a result of the dominant enhancement from and the fill factor, the overall distribution of the energy conversion efficiency of the nanoparticle-integrated solar cells increases from 8.40% to 9.25%, a 10% increase, as shown in Fig. 6(d).
To identify the light-trapping effect of the nanoparticles, the spectrum response from the EQE measurement is presented in Fig. 7(a) for the best-performing plasmonic solar cells in terms of energy conversion efficiency. A broadband light-trapping effect from 550 to 800 nm is observed because of the integration of the lumpy nanoparticles, as can be seen from the EQE enhancement data in the inset of Fig. 7(a). The characteristics of the same solar cell compared to the reference solar cell are shown in Fig. 7(b), in which an obvious enhancement from 14.5 to and a fill factor enhancement from 64.3 to 67.0 are observed, respectively, due to the nanoparticle incorporation. As a result, the highest achieved energy conversion efficiency of the plasmonic solar cell is 9.5%, which is 12% higher than the reference solar cell. In Fig. 7(b), the curve of the entire minimodule is also presented. Due to the variation of different cells, the efficiency (9.25%) is slightly lower than that of the best-performing solar cell. However, it is still significantly higher than those solar cells without nanoparticle integration.
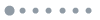
Figure 7.(a) Measured EQE curve of the highest efficiency solar cell with nanoparticles compared to a reference solar cell without nanoparticles. Inset: enhancement of EQE. (b) Current density versus voltage curves for the highest efficiency solar cell with nanoparticles and the nanoplasmonic module compared to a reference solar cell without nanoparticles.
4. CONCLUSION
In conclusion, we have developed innovative and effective nanophotonics methods to tackle the key challenges with the current nanoplasmonic solar cells facilitating the transformation of the light management concept to the viable technology for solar industry. In particular, we demonstrated successful integration of silver lumpy nanoparticles with a large-scale a-Si thin-film solar minimodule. A minimum coverage density of 5%, which is far less than those previously reported, has been found to be the optimized value for the best solar cell performance enhancement due to the superior scatting properties of the lumpy nanoparticles and their uniform distribution over the entire module area. An obvious enhancement in and the fill factor has been observed, which leads to more than a 12% improvement in the energy conversion efficiency of the best solar cell. The results reported in this paper are of great significance for scaling up the plasmonic solar cells toward practical applications.
References
[1] H. A. Atwater, A. Polman. Plasmonics for improved photovoltaic devices. Nat. Mater., 9, 205-213(2010).
[2] J. Springer, A. Poruba, L. Mullerova, M. Vanecek, O. Kluth, B. Rech. Absorption loss at nanorough silver back reflector of thin-film silicon solar cells. J. Appl. Phys., 95, 1427-1429(2004).
[3] H. R. Stuart, D. G. Hall. Island size effects in nanoparticle-enhanced photodetectors. Appl. Phys. Lett., 73, 3815-3817(1998).
[4] V. E. Ferry, J. N. Munday, H. A. Atwater. Design considerations for plasmonic photovoltaics. Adv. Mater., 22, 4794-4808(2010).
[5] M. Gu, Z. Ouyang, B. Jia, N. Stokes, X. Chen, N. Fahim, X. Li, M. J. Ventura, Z. R. Shi. Nanoplasmonics: a frontier of photovoltaic solar cells. J. Nanophoton., 1, 235-248(2012).
[6] S. Xie, Z. Ouyang, B. Jia, M. Gu. Large-size, high uniformity, random silver nanowire networks as transparent electrodes for crystalline silicon wafer solar cells. Opt. Express, 21, A355-A362(2013).
[7] F. J. Beck, S. Mokkapati, K. R. Catchpole. Plasmonic light-trapping for Si solar cells using self-assembled Ag Nanoparticles. Prog. Photovoltaics, 18, 500-504(2010).
[8] Z. Ouyang, S. Pillai, F. J. Beck, O. Kunz, S. Varlamov, K. R. Catchpole, P. Campbell, M. A. Green. Effective light trapping in polycrystalline silicon thin-film solar cells by means of rear localized surface plasmons. Appl. Phys. Lett., 96, 261109(2010).
[9] X. Chen, B. H. Jia, J. K. Saha, B. Y. Cai, N. Stokes, Q. Qiao, Y. Q. Wang, Z. R. Shi, M. Gu. Broadband enhancement in thin-film amorphous silicon solar cells enabled by nucleated silver nanoparticles. Nano Lett., 12, 2187-2192(2012).
[10] N. Fahim, Z. Ouyang, Y. N. Zhang, B. H. Jia, Z. R. Shi, M. Gu. Efficiency enhancement of screen-printed multicrystalline silicon solar cells by integrating gold nanoparticles via a dip coating process. Opt. Mater. Express, 2, 190-204(2012).
[11] Y. N. Zhang, Z. Ouyang, N. Stokes, B. H. Jia, Z. R. Shi, M. Gu. Low cost and high performance Al nanoparticles for broadband light trapping in Si wafer solar cells. Appl. Phys. Lett., 100, 151101(2012).
[12] D. M. Schaadt, B. Feng, E. T. Yu. Enhanced semiconductor optical absorption via surface plasmon excitation in metal nanoparticles. Appl. Phys. Lett., 86, 063106(2005).
[13] D. Derkacs, S. H. Lim, P. Matheu, W. Mar, E. T. Yu. Improved performance of amorphous silicon solar cells via scattering from surface plasmon polaritons in nearby metallic nanoparticles. Appl. Phys. Lett., 89, 093103(2006).
[14] J. L. Wu, F. C. Chen, Y. S. Hsiao, F. C. Chien, P. L. Chen, C. H. Kuo, M. H. Huang, C. S. Hsu. Surface plasmonic effects of metallic nanoparticles on the performance of polymer bulk heterojunction solar cells. ACS Nano, 5, 959-967(2011).
[15] N. F. Fahim, B. H. Jia, Z. R. Shi, M. Gu. Simultaneous broadband light trapping and fill factor enhancement in crystalline silicon solar cells induced by Ag nanoparticles and nanoshells. Opt. Express, 20, A694-A705(2012).
[16] V. E. Ferry, M. A. Verschuuren, M. C. Van Lare, R. E. I. Schropp, H. A. Atwater, A. Polman. Optimized spatial correlations for broadband light trapping nanopatterns in high efficiency ultrathin film a-Si:H solar cells. Nano Lett., 11, 4239-4245(2011).
[17] T. L. Temple, G. D. K. Mahanama, H. S. Reehal, D. M. Bagnall. Influence of localized surface plasmon excitation in silver nanoparticles on the performance of silicon solar cells. Sol. Energy Mater. Sol. Cells, 93, 1978-1985(2009).
[18] N. Fahim, Z. Ouyang, B. Jia, Y. Zhang, Z. Shi, M. Gu. Enhanced photocurrent in crystalline silicon solar cells by hybrid plasmonic antireflection coatings. Appl. Phys. Lett., 101, 261102(2012).
[19] B. Cai, N. Stokes, B. Jia, M. Gu. Near-field light concentration of ultra-small metallic nanoparticles for absorption enhancement in a-Si solar cells. Appl. Phys. Lett., 102, 093107(2013).
[20] W. Yan, N. Stokes, B. Jia, M. Gu. Ag nanocones enhanced plasmonic light trapping in the silicon substrate. Opt. Lett., 38, 395-397(2013).
[21] X. Chen, B. H. Jia, J. K. Saha, N. Stokes, Q. Qiao, Y. Q. Wang, Z. R. Shi, M. Gu. Strong broadband scattering of anisotropic plasmonic nanoparticles synthesized by controllable growth: effects of lumpy morphology. Opt. Mater. Express, 3, 27-34(2013).
[22] N. Stokes, B. H. Jia, M. Gu. Design of lumpy metallic nanoparticles for broadband and wide-angle light scattering. Appl. Phys. Lett., 101, 141112(2012).
[23] J. B. Lassiter, H. Sobhani, J. A. Fan, J. Kundu, F. Capasso, P. Nordlander, N. J. Halas. Fano resonances in plasmonic nanoclusters: geometrical and chemical tunability. Nano Lett., 10, 3184-3189(2010).
[24]
[25] T. L. Temple, G. D. K. Mahanama, H. S. Reehal, D. M. Bagnall. Influence of localized surface plasmon excitation in silver nanoparticles on the performance of silicon solar cells. Sol. Energy Mater. Sol. Cells, 93, 1978-1985(2009).
[26] X. Chen, B. Jia, Y. Zhang, M. Gu. Breaking the limit of plasmonic light trapping in textured screen-printed solar cells using Al nanoparticles and wrinkle-like graphene sheets. Light Sci. Appl..
[27] Y. Zhang, X. Chen, H. Lu, Z. Ouyang, B. Jia, M. Gu. Improved multicrystalline Si solar cells by light trapping from Al nanoparticle enhanced antireflection coating. Opt. Mater. Express, 3, 489-495(2013).