
- Chinese Optics Letters
- Vol. 22, Issue 3, 031304 (2024)
Abstract
1. Introduction
Microwave photonics was proposed to utilize the advantages of photonics technology, including large bandwidth, flexible reconfiguration, and electromagnetic interference (EMI) immunity[1–3]. Up to now, microwave photonic technology has been verified and developed in discrete devices based on optical fiber and RF link cable, and it has shown significant progress. However, such microwave photonic systems are generally bulky in volume and lack stability. With the rapid development of photonic integration, integrating core optoelectronic devices onto the chip to form chip-scale microsystems is considered as a feasible technological route[4]. Currently, integrated microwave photonic signal processing microsystems have been explored on three main integrated optical material platforms: silicon-on-insulator (SOI)[5,6], silicon nitride (
A typical microwave photonic signal processing system includes light sources, modulators, optical filters, and detectors. As essential electronic-optic conversion devices, the properties of modulators affect the performance of microwave photonic systems. For instance, the bandwidth of the modulator determines the upper limit of the working bandwidth of the system. The linearity of the modulator affects the dynamic range of the system. Optical filters are generally adopted to achieve sideband selection or suppression in the optical domain[21]. For example, in a typical microwave frequency measurement system, the relationship of optical power and microwave frequency is established by measuring the output power change of optical filters[22]. In the microwave photonic mixing system, optical filters are used to suppress mixing spurs[23,24]. The performance of the filter affects the quality of sideband selection. In order to enhance the frequency selectivity and out-of-band suppression effect, tunable filters with high extinction ratio and near-rectangular filtering response are always required.
In this work, we report a microwave photonic sideband selector based on the TFLN platform. The device mainly consists of a wideband asymmetrical electro-optic Mach–Zehnder modulator (MZM) and a thermo-optic tunable flat-top microring filter with GHz bandwidth and high out-of-band suppression ratio. Due to the high performance of components of the microwave photonic sideband selector, it supports working bandwidth up to 40 GHz, with undesired sidebands effectively suppressed by more than 25 dB. The related design methods and principles have been reported by our research team previously[25–28]. The insertion loss of a packaged microwave photonic sideband selector is approximately 11 dB, occupying a volume of
Sign up for Chinese Optics Letters TOC. Get the latest issue of Chinese Optics Letters delivered right to you!Sign up now
2. Design and Simulation
The schematic diagram of the proposed TFLN microwave photonic sideband selector is shown in Fig. 1(a). The device is designed on the 500-nm-thick X-cut TFLN wafer. It consists of multimode interferometers (MMIs), spot-size converters (SSCs), an asymmetric electro-optic MZM, and a thermo-optic tunable flat-top microring filter. For the convenience of testing, an asymmetric MZM is adopted in the design. The electro-optic MZM is driven by a 0.9-cm-long ground–signal–ground (GSG) traveling-wave electrode working in a push–pull mode. The central wavelength of the passband of the filter is adjustable utilizing the thermo-optic effect of LN to select the target sideband generated by electro-optic modulation. In addition, spot-size converters are adopted for chip-to-fiber coupling. Two 50:50 MMIs are used as symmetrical optical power splitters and combiners.
Figure 1.(a) Schematic diagram of TFLN microwave photonic sideband selector. (The schematic is not drawn to scale.) (b) Cross-sectional view of the electro-optic modulation region and the thermo-optic tunable filter; (c) definition of design parameters of the microring filter.
The cross-sectional view of the electro-optic modulation region is shown in Fig. 1(b). By reasonably setting the electrode parameters, waveguide width, and etching depth, an excellent electro-optic performance can be achieved. The LN ridge waveguide has a width of
Figure 2(a) shows the operating principle of the proposed device. The target sideband generated by electro-optical modulation is purposefully filtered out by the tunable filter, while the optical carrier and the other sidebands are suppressed. The system simulations of the microwave photonic sideband selector were performed using integrated circuit solver Lumerical INTERCONNECT. The continuous-wave (CW) laser is used with a central frequency of 193.1 THz and output power of 10 dBm. The DC bias voltage is 1.37 V. The frequency of the input sine signal is 40 GHz, with normalized amplitude set to 0.5. The sample rate of the simulation is 0.16 THz, and the time window is
Figure 2.(a) Working principle of the proposed microwave photonic sideband selector. CWL, continuous wave laser; MZM, Mach–Zehnder modulator; TF, tunable filter; OSA, optical spectrum analyzer. (b) Simulated dual sideband modulation; (c) simulated sideband selection.
3. Device Fabrication and Measurement
All involved devices are fabricated on a commercial TFLN wafer (purchased from NANOLN). The patterns are defined using EBL (Vistec EPBG5000+) and inductively coupled plasma (ICP). Chrome is used as a hard mask for etching. The silicon dioxide cladding layer is deposited onto the LN layer by plasma-enhanced chemical vapor deposition (PECVD). The modulation electrodes and thermo-optic microheaters are deposited by electron-beam evaporation (EBE) and lift-off processes. The chemical mechanical polishing (CMP) treatment is carried out to make the end face of the spot-size converters smooth. The optical coupling between the LN waveguide and the polarization-maintaining fiber array (mode field diameter is 3.5 µm) is achieved by UV curing adhesive. For electrical packaging, a ceramic submount for the transmission connection of the RF signals is used. In order to minimize RF loss and reflection, a specific RF link is designed, including RF transmission lines mounted on ceramics to carry the driving signals from 1.85 mm coaxial connectors and from modulators to an off-chip matching resistor (38 Ω).
The electro-optic responses of the TFLN asymmetric MZM is characterized by using a vector network analyzer (Ceyear, 3672E). As is shown in Fig. 3(a), the measured electro-optic 3 dB bandwidth is about 50 GHz, which determines the upper limit of working bandwidth of the proposed device. A continuous tunable laser (Santec, TLS-510), an optical power meter (AQ2211, Yokogawa), and a programming computer constitute the wavelength scanning system to measure the optical transmission spectrum of the TFLN filter. As is shown in Fig. 3(b), the filter has a 3 dB bandwidth of about 4.8 GHz and an out-of-band rejection ratio of 33.48 dB. The free spectral range (FSR) is about 1.6 nm.
Figure 3.(a) Normalized electro-optic responses of the TFLN asymmetric MZM; (b) measured bandpass filtering response of the TFLN filter.
The image of the packaged microwave photonic sideband selector is shown in Fig. 4(a). The packaged device occupies a volume of
Figure 4.(a) Image of the packaged microwave photonic sideband selector; (b) optical transmission spectrum of the microwave photonic sideband selector. The illustration is a partially enlarged view of the optical transmission spectrum of the microwave photonic sideband selector.
Finally, the function of sideband selection is evaluated. A sine signal at 40 GHz of 10 dBm is generated by the signal generator (Keysight, N51738) and sent into the microwave photonic sideband selector. The optical carrier with a central frequency of 193.10 THz with output power of 0 dBm is provided by a continuous tunable laser (Santec, TSL-510). The precision DC power supply (Keysight, B2901A) is used to adjust the central wavelengths of the filters. For the asymmetrical electro-optic MZM, the electro-optic modulation effect is similar to dual sideband modulation, with relatively low modulation efficiency. Figure 5(a) exhibits the electro-optic modulation optical spectra after the asymmetrical MZM. The positive and negative first-order sidebands and higher-order sidebands are generated. In the experiment, the target sideband is a positive first-order sideband. Subsequently, the central frequency of the filter is adjusted to align with the positive first-order sideband. As shown in Fig. 5(b), the positive first-order sideband is filtered out, while the optical carrier and negative first-order sideband are suppressed by 25 dB.
Figure 5.(a) Measured optical spectra after asymmetrical MZM; (b) measured optical spectra of sideband selection of the demonstrated TFLN microwave photonic sideband selector.
4. Conclusions
In conclusion, we first propose and demonstrate a monolithic microwave photonic sideband selector based on the TFLN platform. The device consists of on-chip spot-size converters, an electro-optic MZM, and a thermo-optic flat-top tunable microring filter. It has achieved two functions: electro-optic modulation of a wideband RF signal and target sideband selection. The microwave sideband selector supports a working bandwidth up to 40 GHz, with undesired sidebands effectively suppressed by more than 25 dB. The packaged microwave photonic sideband selector exhibits a compact volume of
References
[2] J. Yao. Microwave photonics. J. Lightwave. Tech., 27, 314(2009).
[4] D. Marpaung, J. Yao, J. Capmany. Integrated microwave photonics. Nat. Photonics, 13, 80(2019).
[19] D. Pohl, F. Kaufmann, M. R. Escalé et al. Tunable Bragg grating filters and resonators in lithium niobate-on-insulator waveguides. CLEO: Science and Innovations, STu4J.5(2020).
[21] A. Mast, C. Middleton, S. Meredith et al. Extending frequency and bandwidth through the use of agile, high dynamic range photonic converters. 2012 IEEE Aerospace Conference, 1(2012).
[23] Z. Tang, S. Pan. Microwave photonic mixer with suppression of mixing spurs. 2015 14th International Conference on Optical Communications and Networks (ICOCN), 1(2015).
[25] X. Wang, C. Shang, A. Pan et al. Thin-film lithium niobate based dual-polarization IQ modulator for single-carrier 1.6 Tb/s transmission. Integrated Optics: Devices, Materials, and Technologies XXVI, 87(2022).
[26] C. Shang, A. Pan, C. Hu et al. 112 Gb/s PAM4 electro-optic modulator based on thin-film LN-on-insulator. Optoelectronic Devices and Integration, OW1B.3(2019).
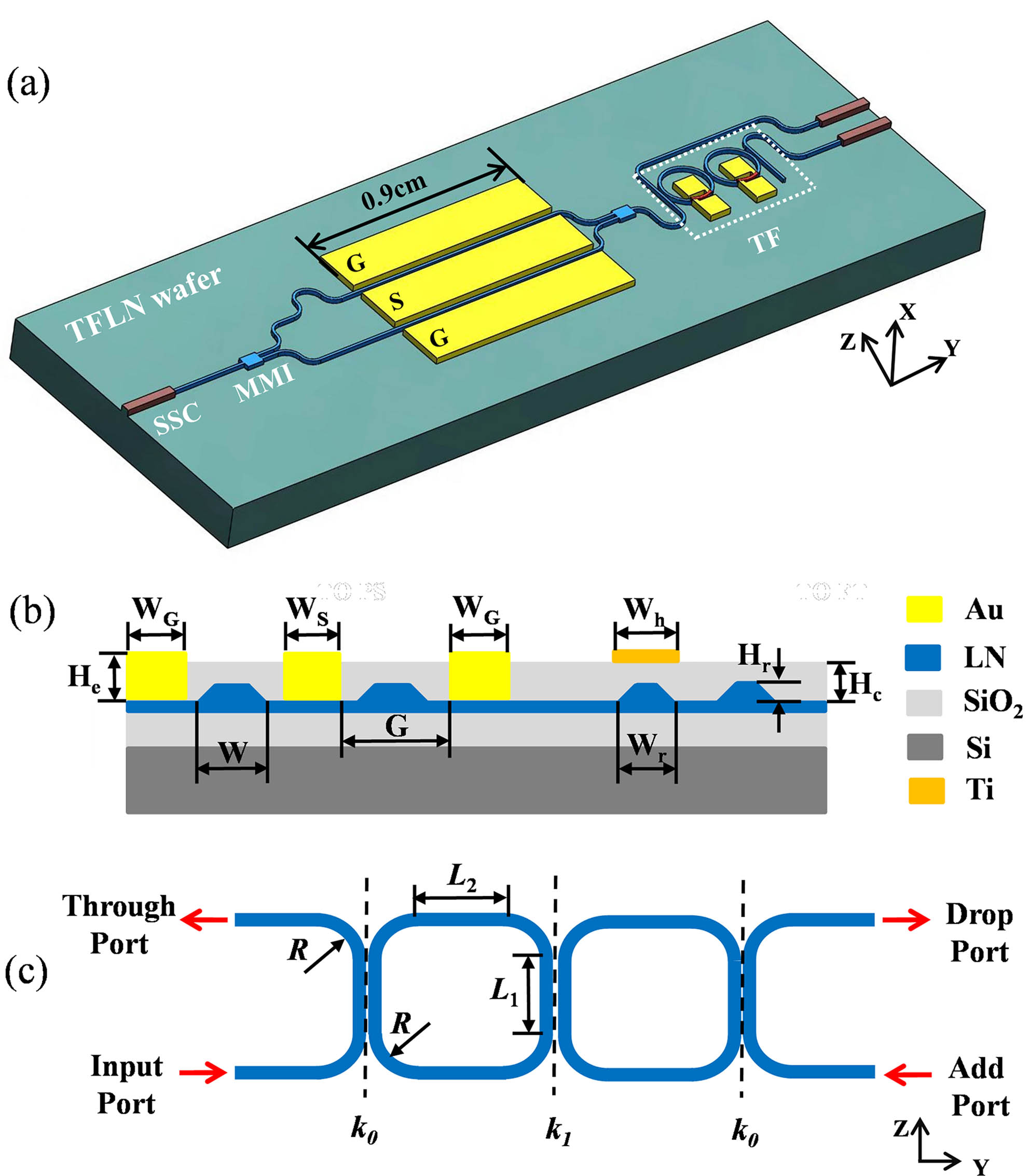
Set citation alerts for the article
Please enter your email address