G. Baishali1、*, V. Radhakrishna2, V. Koushal1、2, K. Rakhee2, and and K. Rajanna1
Abstract
The detection efficiency of a gaseous photomultiplier depends on the photocathode quantum efficiency and the extraction efficiency of photoelectrons into the gas. In this paper we have studied the performance of an UV photon detector with P10 gas in which the extraction efficiency can reach values near to those in vacuum operated devices. Simulations have been done to compare the percentage of photoelectrons backscattered in P10 gas as well as in the widely used neon-based gas mixture. The performance study has been carried out using a single stage thick gas electron multiplier (THGEM). The electron pulses and electron spectrum are recorded under various operating conditions. Secondary effects prevailing in UV photon detectors like photon feedback are discussed and its effect on the electron spectrum under different operating conditions is analyzed.1. INTRODUCTION
A cesium iodide photocathode coupled to an electron multiplying structure like the thick gas electron multiplier (THGEM) can act as a gaseous photomultiplier (GPM) sensitive to UV light [1,2]. The advantage of GPM is that it can achieve a very large active area with moderate position and timing resolution [3]. In semitransparent (ST) configuration, a thin film of CsI (30 nm) is coated over an UV transparent quartz window that acts as the photocathode. This is mounted a few millimeters above the electron multiplier.
A schematic representation of the working principle of a ST-type photocathode is shown in Fig 1. UV photons hitting the photocathode will emit a photoelectron that under the influence of the electric field moves toward the THGEM. These electrons experience a high electric field across the THGEM hole and produce avalanche multiplication even up to . In effect, a single electron produced by an UV photon can be detected by this.

Figure 1.UV photon detector working in ST configuration.
During the transit of the photoelectrons from the photocathode surface through the gas medium, some fraction of photoelectrons are scattered back to the photocathode surface as a result of elastic collisions with the gas atoms/molecules, even in the presence of the electric field. This effect results in a noticeable degradation of the effective photoemission from the photocathode in a gas medium as compared to vacuum [2–5], leading to reduction of the GPM detection efficiency.
Sign up for Photonics Research TOC. Get the latest issue of Photonics Research delivered right to you!Sign up now
There is various literature in which UV photon detection has been done with CsI photocathode coupled to THGEM [4,5]. But most of them are in a reflective mode, in which the CsI is directly deposited over the THGEM top electrode. The main advantage of using this configuration is the absence of the photon feedback effect, which restricts the performance of an UV photon detector. However, the effective photocathode area is more in the case of a ST configuration compared with a reflective one. Also, the ion backflow in cascaded THGEM GPMs coupled to a ST photocathode is as low as 2%, whereas for the reflective configuration, it is [6]. Thus, ST configuration can be considered for UV photon detection application.
To our knowledge, there was no literature available that studied the THGEM performance as an UV photon detector in ST mode with P10 gas mixture, which was considered a good gas mixture in terms of reduced backscattering [1,7]. It has been reported that the detection efficiency of GPMs using mixture reaches values more than 50% of that obtained in vacuum-operated devices under proper electric field conditions [1,8]. In [7], it is reported for mixture, the gas to vacuum photocurrent ratio (i.e., the relative extraction efficiency) is at an electric field of . Argon-based mixtures, which are considered as non-ageing mixtures [9], are also used in gas electron multiplier (GEM)-based GPMs [10].
In this paper, initially we have estimated the backscattering percentage in P10 gas and compared it with the widely used neon-based gas mixture. The performance of the UV photon detector in P10 mixture was studied experimentally. The electron pulse and the electron spectra were recorded and analyzed to find how the secondary effects like photon feedback affects the electron spectrum in an UV photon detector.
2. MODELING AND SIMULATION
In order to get an estimate of the percentage of photoelectrons backscattered to the photocathode, we have used the Monte Carlo technique with GARFIELD [11] to simulate the performance of the detector. 3D finite element modeling along with the electric field computation of the THGEM acting as the electron multiplier was carried out using ANSYS. The electron generation and transport properties in various gas mixtures were simulated using the GARFIELD program. The steps of simulation are described in [12].
Simulation results show that at 1 atm, the backscattering losses in P10 gas is around 18%, whereas for () it is . We have also estimated this percentage in another neon-based mixture, (), widely used for UV photon detector applications. In this mixture, the backscattered photoelectrons percentage is . The simulations had been carried out for a drift field of .
Thus, from the simulation results, it can be concluded that P10 can also be considered as a good gas for UV photon detectors in terms of backscattering phenomenon.
3. EXPERIMENTAL PROCEDURE
A. THGEM Used as the Electron Multiplier Element
THGEMs having insulator thicknesses of 400 to 1000 μm, hole pitches between 700 and 1000 μm, and diameters between 300 and 1000 μm are typically used for UV photon detector applications [4,13]. In our studies presented in this paper, the geometrical parameters of the THGEM used were insulator thickness 250 μm, hole diameter 200 μm, and pitch 550 μm. A 100 μm rim created by chemical etching of copper was provided around each hole for reducing the probability of discharges [13].
B. Thermal Evaporation of CsI
CsI photocathode film was deposited onto the chromium-coated quartz using the thermal evaporation technique. In thermal evaporation, the material to be deposited is usually taken in the form of a powder in a molybdenum boat, which can withstand high temperature. The thermal evaporation process was carried out inside a vacuum chamber. High-purity cesium iodide (99.995% purity) was taken as the starting material. The melting point of CsI at is 621°C. The film deposition was done under a vacuum of . The deposition rate was maintained at 1–2 nm/s. The thickness of the deposited CsI for ST photocathode is around 30 nm. This is the thickness typically used in literature for ST configuration [13]. The samples were annealed under high vacuum at 70°C for 4 h to enhance the photoemission from the photocathode [14].
The UV transmission of the substrate used for a photocathode was measured with a spectrophotometer as shown in Fig. 2. The substrate (quartz with the chromium layer) shows a transmission of around 50% at a wavelength of 200 nm.
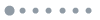
Figure 2.Transmission curve for chromium coated quartz.
C. Experimental Setup
The test chamber used for this study was made of aluminum with the dimensions of . The readout electrode (copper strips etched from a PCB sheet) was placed first in the mounting assembly. The required induction gap, i.e., the gap between the THGEM bottom electrode and the readout electrode, was maintained using spacers of different heights. The top plate was made up of PERSPEX material having an arrangement for holding the photocathode few millimeters above from the THGEM structure. The electronic chain used for acquiring the spectrum is shown in Fig. 3. A low pressure Hg lamp emitting in the range of 100–280 nm was used as a UV source for our measurements.
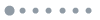
Figure 3.Schematic representation of the electronic chain used for recording the electron spectra from the UV photon detector.
4. RESULTS AND DISCUSSION
A. Study of the Electron Pulse and Electron Spectra from the UV Photon Detector
Electron spectra from the single THGEM-based GPM was acquired under different operating conditions, and detector properties like secondary effects prevailing at various operating conditions were studied. The spectra were taken in pulse-counting mode. The charge pulse is collected from the readout strips through a charge-sensitive preamplifier (ORTEC 142PC). The preamplifier converts the charge output from the detector to voltage output. Output signal of the preamplifier has a fast rise time and long fall time. A shaping amplifier (ORTEC 671A) was used for shaping the signal pulse from the preamplifier. The output of the amplifier was fed to a multichannel analyzer (MCA8000A). The detector pulse was observed in a digital oscilloscope (Tektronix DPO 4104) and the electron spectrum was recorded using the PC.
For our study, it was assumed that the number of photoelectrons generating a single signal pulse of a given pulse height remains the same throughout the present study. A drift gap of 5 mm was maintained in these studies. Figure 4 shows the output from the oscilloscope for a pin-hole aperture () placed in front of the UV light source. The pulses captured in the oscilloscope with amplitude of , corresponds to preamplifier output, which is caused by few hundred photoelectrons. was maintained at 1250 V for this experiment. Based on the measurement done for X-rays, at of 1250 V the expected gain would be . The effective gain measurements have been studied using a fine collimated source of X-ray ( source) shined over the THGEM through the aluminized Mylar window.
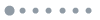
Figure 4.Electron pulses as seen in the oscilloscope for pin-hole aperture.
Electron spectra recorded for fixed drift and induction field with various are shown in Fig. 5. The acquisition time was kept constant at 10 min. The drift and induction fields were maintained at in all the cases. P10 gas at 980 mbar pressure was used for the study. The lower portion of the spectrum merges with the electronic noise. The recorded spectrum is due to multiple electrons, which are expected to have a peak with the higher portion of the curve following an exponentially decreasing shape [15]. Any deviation from the exponential behavior is expected to be related to secondary effects like photon feedback. The slope of the curve in the straight-line region indicates the gain at that particular THGEM voltage, which increases with the increase of .
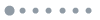
Figure 5.Electron spectra for different multiplication voltages recorded with a MCA.
The slope of the curve was estimated at the central portion of the spectrum. This was done to exclude any error in the estimation coming from the electronic noise contribution in the lower part of the spectrum or from the feedback effects at the higher portion of the spectrum.
5. EFFECT OF PHOTON FEEDBACK ON ELECTRON SPECTRA
Analysis of the electron spectra obtained from the GPM working in the UV spectral range shows that the spectrum deviates from the exponential nature at higher channel numbers of the MCA. The deviation is caused due to the presence of some excess electrons contributing to the pulse height in this region of the spectrum. The presence of excess pulses as an enhanced tail of the exponential distribution in the spectrum is a clear indication of photon feedback [16]. A brief account of these phenomena is given below.
During high electron multiplication inside the THGEM hole, in addition to ionization, there may be simple excitation of the gas molecules without creation of secondary electrons. These excited molecules decay directly to the ground state through the emission of a visible or UV photons [17]. Some of these photons may escape the absorption by the quenching gas and hit back the photocathode emitting photoelectrons, which contribute to the pulse height. This is known as photon feedback effect [18]. This effect results significantly in the photocathode aging and hence is considered a major performance limiting factor for UV photon detectors.
We have studied the effect of different operating parameters like and drift field on photon feedback.
A. Effect of ΔVTHGEM on Photon Feedback
In order to study the effect of on the photon feedback, the tail portion of the spectrum was analyzed. The deviation of the curve from an exponential distribution in Fig. 5 increases with . This indicates that photon feedback increased with . Also the number of secondary pulses show an increase at high compared to lower . This can be seen clearly in Fig 6. This figure is the zoomed view of the tail-end portion of curves shown in Fig. 5. The observation can be explained as follows. As is increased, the gain inside the THGEM hole increases, thus increasing the probability of photon feedback. This results in increased secondary photoelectron emission contributing to the signal pulse height. The problem of photon feedback can be minimized if the detector is operated in the multi-THGEM configuration, keeping the gain across the first THGEM element low (by maintaining a low multiplication voltage across the first THGEM). Because of low optical transparency of the THGEM (), the photon feedback to the photocathode from the high gain second or third stage will be low [18].
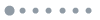
Figure 6.Excess pulses due to photon feedback at different .
B. Effect of Drift Field on Secondary Effects
We also studied the effect of the drift field, i.e., the electric field present between the photocathode and the THGEM on photon feedback at a of 1235 V. The study has been carried out for three different drift fields of , , and . In all the cases, the induction field was maintained at . P10 gas at 980 mbar was used. As shown in Fig. 7, it is observed that the feedback effect is more pronounced at lower drift field of as compared to higher drift field of . This observation was valid for other of 1150 and 1200 V also (not shown here). The observation can be explained as follows. At lower drift fields, the transfer efficiency of photoelectrons from the photocathode surface to the THGEM hole is more compared to that at higher drift fields [12]. Simulation results show that the electron transfer efficiency at is , whereas at and they are around 85% and 75%, respectively. The steps of simulation are described in [11]. Because of higher transfer efficiency at lower drift field, more electrons reach the GEM hole for multiplication, giving higher effective gain for each pulse. Also, having more electrons subjected to multiplication enhances the probability of generation of secondary photons at lower drift field. But at a higher drift field, even though the feedback effect is reduced, the detection efficiency of the detector will also reduce due to lesser number of electrons reaching the hole for multiplication. However, this problem can be solved by using THGEM in multistage configuration to increase the overall gain of the detector without increasing the probability of photon feedback.
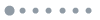
Figure 7.Effect of drift field on photon feedback.
6. CONCLUSIONS
The detection efficiency of GPMs depends on the photocathode quantum efficiency and the extraction efficiency of photoelectrons into the gas medium. P10 gas is considered a good gas in terms of reduced backscattering, which is a major requirement of UV photon detectors. Simulation results show the percentage of backscattered electrons in P10 gas is either less [in comparison to the () mixture] or almost similar to () mixture.
UV photons were successfully detected from a CsI-based photocathode in ST configuration in P10 mixture. The study has been carried out using a single THGEM as the electron multiplier. The electron spectra recorded showed an exponentially decreasing behavior at low . At higher , deviation from the exponential nature was observed at the higher portion of the spectrum. The deviation is due to the presence of excess pulses at the tail portion of the spectrum indicating the generation of secondary pulses due to photon feedback. The number of these secondary pulses showed an increase with increase in .
Study on the effect of drift field on the photon feedback reveals that photon feedback is more pronounced at lower drift field as compared to higher drift field. The effect is correlated with the electron transfer efficiency of the THGEM, which is higher at lower drift fields.
References
[1] A. Breskin, M. Balcerzyk, R. Chechik, G. P. Guedes, J. Maia, D. Mörmann. Recent advances in gaseous imaging photomultipliers. Nucl. Instrum. Methods Phys. Res. A, 513, 250-255(2003).
[2] A. Breskin, T. Boutboul, A. Buzulutskov, R. Chechik, G. Garty, E. Shefer, B. K. Singh. Advances in gas avalanche photomultipliers. Nucl. Instrum. Methods Phys. Res. A, 442, 58-67(2000).
[3] T. Sumiyoshi, F. Tokanai, H. Sugiyama, T. Okada, N. Ohishi, T. Ohmura, H. Sakurai, S. Gunji. Development of a gaseous PMT with micro-pattern gas detectors. Nucl. Instrum. Methods Phys. Res. A, 639, 121-125(2011).
[4] C. D. R. Azevedo, M. Cortesi, A. V. Lyashenko, A. Breskin, R. Chechik, J. Miyamoto, V. Peskov, J. Escada, J. F. C. A. Veloso, J. M. F. dos Santos. Towards THGEM UV-photon detectors for RICH: on single-photon detection efficiency in Ne/CH4 and Ne/CF4. JINST, 5, P01002(2010).
[5] H.-B. Liu, Y.-H. Zheng, Y.-G. Xie, J.-G. Lu, L. Zhou, B.-X. Yu, A.-W. Zhang, Z.-H. An, Y.-G. Xie, D. Zhang, Z.-P. Zheng. Study of the THGEM detector with a reflective CsI photocathode. Chin. Phys. C, 35, 363-367(2011).
[6] R. Chechik, A. Breskin. Advances in gaseous photomultipliers. Nucl. Instrum. Methods Phys. Res. A, 595, 116-127(2008).
[7] A. F. Buzulutskov. Gaseous photodetectors with solid photocathodes. Phys. Part. Nucl., 39, 424-453(2008).
[8] A. Breskin, A. Buzulutskov, R. Chechik. GEM photomultiplier operation in CF4. Nucl. Instrum. Methods Phys. Res. A, 483, 670(2002).
[9] A. Buzulutskov, A. Breskin, R. Chechik, G. Garty, F. Sauli, L. Shekhtman. The GEM photomultiplier operated with noble gas mixtures. Nucl. Instrum. Methods Phys. Res. A, 443, 164-180(2000).
[10] A. Breskin, A. Buzulutskov, R. Chechik, B. K. Singh, A. Bondar, L. Shekhtman. Sealed GEM photomultiplier with a CsI photocathode: ion feedback and ageing. Nucl. Instrum. Methods Phys. Res. A, 478, 225-229(2002).
[11]
[12] G. Baishali, V. Radhakrishna, V. Koushal, K. Rakhee, K. Rajanna. Study of electron focusing in thick GEM based photon detectors using semitransparent photocathodes. Nucl. Instrum. Methods Phys. Res. A, 729, 51-57(2013).
[13] C. Shalem, R. Chechik, A. Breskin, K. Michaeli. Advances in Thick GEM-like gaseous electron multipliers—Part I: atmospheric pressure operation. Nucl. Instrum. Methods Phys. Res A, 558, 475-489(2006).
[14] H. Rabus, U. Kroth, M. Richter, G. Ulm, J. Friese, R. Gernhäuser, A. Kastenmüller, P. Maier-Komor, K. Zeitelhack. Quantum efficiency of cesium iodide photocathodes in the 120–220 nm spectral range traceable to a primary detector standard. Nucl. Instrum. Methods Phys. Res. A, 438, 94-103(1999).
[15] F. Sauli. Novel Cherenkov photon detectors. Nucl. Instrum. Methods Phys. Res. A, 553, 18-24(2005).
[16] D. Mörmann, A. Breskin, R. Chechik, B. K. Singh. On the efficient operation of a CsI-coated GEM photon detector. Nucl. Instrum. Methods Phys. Res. A, 471, 333-339(2001).
[17] G. F. Knoll. Radiation Detection and Measurement(1989).
[18] A. Buzulutksov. Radiation detectors based on gas electron multipliers. Instrum. Exp. Tech., 50, 287-310(2007).