
- Chinese Optics Letters
- Vol. 22, Issue 3, 030602 (2024)
Abstract
1. Introduction
The transmission capacity of single-mode optical fibers has nearly approached the Shannon limit[1]. In order to meet the demands of high-capacity communication networks, various multiplexing techniques have been proposed, such as wavelength-division multiplexing (WDM)[2–4], polarization-division multiplexing (PDM)[5], and spatial-division multiplexing (SDM)[6–9]. Over the past few decades, WDM has played an important role in mode-division multiplexing (MDM) technology based on few-mode fiber (FMF), which is a form of SDM and presents a promising solution to the capacity crisis. An MDM communication system contains orthogonal modes of different orders such as linearly polarized (LP)[10,11] and orbital angular momentum (OAM)[12–14] modes as independent channels within the FMFs. Recently, various devices for MDM communication systems have been proposed. These include mode multiplexers/demultiplexers (MMUXs/MDEMUXs), high-order mode fibers[8], mode-selective switches, and so on. MMUX/MDEMUX devices constitute the cornerstone of the MDM communication system and have been extensively researched. This encompasses various types of devices, including photonic-lantern-based multiplexers[15], waveguide devices[16], diffraction devices[17], liquid crystals[18], and so on. The data exchange between different channels is an indispensable function in the optical communication network. The traditional mode exchange approach initially demultiplexes multiple orthogonal mode lights into fundamental mode lights, performs mode exchange, and then remultiplexes using an MMUX, which involves multiple devices with a wide footprint and is inconsistent with the trend toward photonic integration. Therefore, it is necessary to propose an integrated mode exchange device. In addition, the integrated mode exchange device can be employed to balance the differential mode delay (DMD) in the long-distance optical fiber communication. DMD is defined as the relative delay of the transmission between mode groups[19]. The increase in DMD leads to a degradation in communication quality, accompanied by an elevation in the complexity of MIMO-DSP at the receiver. The data exchange between different mode groups is an effective solution for reducing the computational cost and minimizing system power consumption. For the device, the number of modes supported, conversion efficiency, and miniaturization are essential concerns. The mode exchange devices based on a silicon metastructure that achieved high conversion efficiencies have been reported[20].
Diffractive optical elements are phase components that generate the desired optical field through interference and diffraction. Metasurface is a technology for achieving efficient phase shift[21]. By combining a metasurface on top of a planar waveguide, efficient conversion between TM and TE modes is achieved with 98% analog mode purity[22]. The researchers combined volume holograms with a random optical diffuser, and low crosstalk mode exchange was obtained[23]. However, the multiple quantity modes and the miniaturization cannot be fulfilled at the same time. Multiplane light conversion (MPLC) devices that utilize a multi-layer diffraction structure composed of multiple phase planes to modulate the input light field were proposed by researchers from CaiLabs in 2014. The MPLC devices have a high degree of freedom in wavefront modulation and demonstrate a unique advantage in mode control[24–27]. In the last few years, low-loss, broadband, and highly mode-selective MMUXs/MDEMUXs based on MPLC have been proposed[26,28]. It is possible to optimize a mode exchange device with low-mode crosstalk and high conversion efficiency using MPLC.
In this paper, we designed and fabricated a mode exchange device for data conversion in optical networks and for balancing DMD in long-distance optical fiber communication. In conjunction with the reported research work on MMUX/MDEMUX based on MPLC in our experiment, phase masks are calculated using a wavefront-matching algorithm. The exchange between input modes (LP01, LP11a, LP11b, and LP21) and target modes (LP11a, LP11b, LP21, and LP01) has been realized. The proposed target can be achieved perfectly with only four phase planes, ensuring simulated mode purity larger than 85%, and this process is reversible. The phase structures were fabricated using photolithography and dry-etching processes on a silicon substrate. In the experiment, we integrated the phase planes and FMFs into a unified package. Notably, this device also serves as a broadband photonic component, capable of spanning the C-band and L-band. The mode exchange device has been successfully applied to MDM communication system, realizing the three-channel modes (LP01, LP11, and LP21) multiplexing and data exchange function. 10 Gbit/s OOK signals and QPSK signals have been successfully demonstrated at the wavelength of 1550 nm. The device supports high-performance data exchange based on an MDM optical communications system, further expanding the versatility and applicability of optical networks.
Sign up for Chinese Optics Letters TOC. Get the latest issue of Chinese Optics Letters delivered right to you!Sign up now
2. Design and Fabrication of the Modes Exchange Device
The modes exchange between multiple coaxial modes, making MDM more balanced during transmission. The modes exchange based on MPLC does not have any mathematical expression. The MPLC is needed to ensure the correspondence between input light field and output light field and modulates the phase shift of the incident light by multiple phase planes. The multiple phase planes are calculated using the wavefront-matching algorithm based on inverse design. The algorithm attempts to match the forward-propagating light field with the backward-propagating light field at any position in space. Thus, the phase distribution of the phase plane is equal to the superposition of the conjugate input light fields and the output light fields in the phase plane. The phase plane is updated by the superposition of the conjugate incident light fields and the output light fields, and the iterative calculation is repeated until the algorithm converges to obtain the multiple phase planes.
Figure 1(a) shows the schematic of modes exchange based on MPLC. The LP01, LP11a, LP11b, and LP21 coaxial modes output from an FMF are exchanged into LP11a, LP11b, LP21, and LP01 coaxial modes when passing through four phase planes. The distance of the incident light field to the first phase plane and the distance between different phase planes are both 20 mm. Figure 1(b) shows the mode exchange sequence. When the LP modes pass through the modes exchange device, LP01 transforms into LP11a, LP11a transforms into LP11b, LP11b transforms into LP21, and LP21 transforms back into LP01 mode, creating a loop. The phase of input (LP01, LP11a, LP11b, and LP21) and target (LP11a, LP11b, LP21, and LP01) light fields are matched by four planes, and phase planes are iteratively updated by the superimposed phases’ convergence. To improve the tolerance of the device, a phase-smoothing factor is incorporated into the design to diminish the phase abruptness of the phase planes. Figure 1(c) shows the multiple phase planes obtained by the algorithm convergence. Based on the design, the phase structures are fabricated on a silicon substrate using photolithography and dry-etching processes with an accuracy of 8 µm. The manufactured phase plane is a reflective device with 16 phase levels, ranging from 0 to
Figure 1.(a) Schematic of modes exchange; (b) mode exchange sequence between LP01, LP11a, LP11b, and LP21 modes; (c) calculated images of the phase planes; (d) microscopic images of the fabricated phase masks.
3. Experimental Results and Analysis
We experimentally demonstrated the LP modes exchange based on inverse design MPLC devices. In the experiment, we used a reflective phase mask and a mirror to build the MPLC setup, which improved module integration and reduced device insert loss (IL). The LP modes (LP01, LP11a, LP11b, and LP21) are generated by a four-mode multiplexer, which also based on MPLC[11]. The LP modes are output from FMF, collimated by a graded index lens (G-lens), and input to the LP modes exchange device. Four coaxial LP modes are reflected back and forth between the gold-coated phase mask and the mirror, and the exchanged modes are output from the LP modes exchange device and coupled to the FMF by another G-lens. The distance between the phase plane and mirror plane is 9.8 mm. The waist diameter of the input beam and output beam is 300 µm. The schematic diagram of the reflective mode exchange device is shown in Fig. 2(a), and the captured physical image is depicted in Fig. 2(b). The IL of the device includes mode conversion loss and reflection loss between the phase plane and mirror. Here, the IL is defined as
Figure 2.(a) Schematic diagram of the reflective mode exchange device; (b) physical image of the device; (c) intensity distribution of the calculated modes; (d) phase distribution of the calculated modes; (e) intensity distribution of the generated modes by the modes exchange device; (f) phase distribution of the reconstructed modes; (g) calculated and (h) experimental mode crosstalk gray matrices.
Figure 3.Conversion efficiency of the mode exchange device over the C-band and the L-band wavelengths.
The mode crosstalk is a phenomenon where different modes interfere with each other and is characterized by the mode crosstalk matrix. The crosstalk between mode
To evaluate the data transmission performances of the mode exchange device, a back-to-back MDM system based on an MMUX, 2 m FMF, and an MDEMUX was characterized for data exchange, as shown in Fig. 4(a). However, LP11a and LP11b modes in the same group within FMF are degenerated modes. They are prone to strong coupling, which affects the effectiveness of communication. Therefore, the LP01, LP11b, and LP21 modes are selected as the three channels for transmission. 10 Gbit/s OOK fundamental mode light signals at the wavelength of 1550 nm generated independently using standard optical transceivers were divided into three groups. The MMUX multiplexed them into the coaxial multimode light signals and then coupled them into FMF. After the transmission of FMF, a mode exchange device is used for data conversion. Finally, the light signals were demultiplexed into three channel fundamental mode light signals by MDEMUX and then detected by the transceiver of the corresponding input channel. The direct detection technique is selected to reduce cost and system complexity. By measuring the input power and output power of the system, the system mode crosstalk gray matrices are obtained, as illustrated in Fig. 4(b), which are below
Figure 4.(a) Experimental setup of the few-mode optical fiber communication system based on mode exchange device; (b) measured mode crosstalk gray matrices of the communication system; (c) measured BER characteristics of the system. Constellation diagrams of the QPSK signals: (d) LP01 mode; (e) LP11 mode; (f) LP21 mode.
For visualization purposes, we also measured the constellation diagrams of three channels (LP01, LP11b, and LP21) carrying 10 Gbit/s QPSK signals. As shown in Figs. 4(d)–4(f), the constellation diagram for the LP21 mode appears slightly more dispersed compared to the other two modes, primarily due to lower received power. The calculated Q-factor exceeds 6.94, which can be further optimized using communication algorithms. Utilizing the G-lens for multimode lights coupling into the FMF during transmission, coupling loss also emerges as a primary contributor to system losses. The combination of mode exchange devices with the MDM communication system enhances the flexibility of optical communication networks. In future research, optimizing the coupling loss and system crosstalk will enable the device to support transmission in long-distance communication.
4. Conclusion
In this paper, we designed and fabricated a mode exchange device for data conversion using MPLC technology in optical networks. The device supports the conversion between input modes (LP01, LP11a, LP11b, and LP21) and target modes (LP11a, LP11b, LP21, and LP01). The mode exchange device has been demonstrated in the MDM communication system. It is worth noting that the method for designing and fabricating the device is scalable. We can configure various input and output mode arrangements to calculate and process different phase structures, thereby enhancing the flexibility of mode conversion. We have demonstrated mode conversion for four modes, and we can showcase mode exchanges for higher-order modes in the future, such as 6-modes or 10-modes. Additionally, we can increase the integration of the device using metasurface technology. The device has exhibited excellent performance in the wideband test, enabling the establishment of the WDM-SDM hybrid systems to enhance communication capacity. Overall, the combination of the mode exchange device, MMUXs/MDEMUXs, and FMFs further increases the capacity of communication systems and enriches the functionality of optical networks.
References
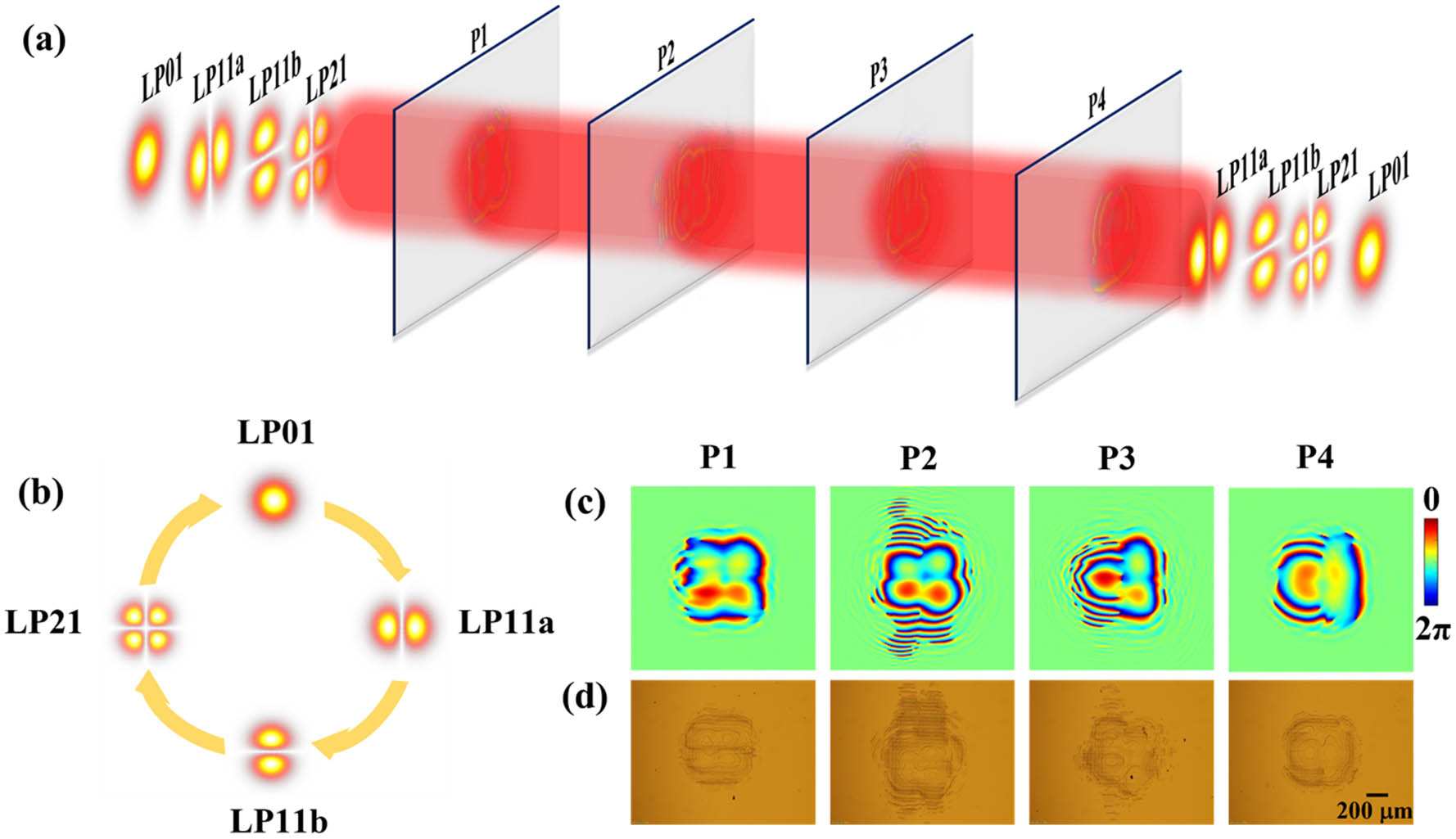
Set citation alerts for the article
Please enter your email address