
- Photonics Research
- Vol. 10, Issue 8, 1892 (2022)
Abstract
1. INTRODUCTION
Visible light communication (VLC) is an attractive optical wireless communication (OWC) technique for short-range communication to meet the growing demand for high-speed wireless connectivity because of its unique features, such as the ability to use an unlicensed spectrum, easy integration with solid-state lighting and, thus, low cost, high modulation bandwidth, as well as high data rate. Moreover, it can be widely used in multiple scenarios, such as indoor positioning, underwater communication, and in-vehicle wireless communication, which can effectively complement radio communication and play an important role in future 6G technology [1]. High-quality optical transmitters, such as LEDs and lasers are one of the core elements for realizing a high-performance VLC system [2]. In recent years, we have seen rapidly increasing advances in the field of VLC based on micro-LEDs, which have demonstrated impressive performances, such as optical bandwidths over 1 GHz [3] and data rates over 10 Gbps [4], thanks to the advantages of good heat dissipation, uniform current spreading, and a small RC constant brought by the small size of the device [5]. However, the small effective luminous area conversely limits its light output power (LOP), which is one of the main obstacles in its application in long-distance communication. To increase the LOP of the micro-LED, researchers have enhanced the quantum efficiency by improving the epitaxy quality and designing the device structure. The special design of the multiple quantum well structure, such as the change in the quantum barrier thickness, can effectively improve the internal quantum efficiency (IQE) or optical bandwidth of the micro-LED [6]. However, the experimental results in Ref. [6] also indicate that there is a trade-off between the LOP and the optical bandwidth under this improved method, and it is difficult to obtain the improvement of both two performances simultaneously. In addition, replacing traditional -plane sapphire with a nonpolar or semipolar substrate has the potential to reduce polarization and enhance radiation recombination, facilitating simultaneous optimization in LOP and bandwidth of the micro-LED. For example, both semipolar blue and green micro-LED arrays have been demonstrated to achieve modulation bandwidths over 1 GHz and LOPs on the order of milliwatts, exhibiting excellent performance in VLC [7,8]. However, it is still limited by the high price of the substrate as well as the lack of good material quality [9].
Excitingly, specially designed parallel or series connection micro-LED structures can relax the trade-off between the LOP and the optical bandwidth as both structures can increase LOP by several times with almost no reduction in bandwidth. On one hand, it has been demonstrated that about 10 times the optical power and roughly the same bandwidth can be obtained compared to a single pixel at the same current density by using a green micro-LED array in parallel [10]. Besides, a similar study has shown that the use of semipolar green micro-LEDs in parallel with a bandwidth of 800 MHz can increase the communication data rate to more than 5 Gbps [11]. On the other hand, a blue micro-LED array in series has also been demonstrated to achieve the LOP over 10 mW and communication performance of 11.74 Gbps/0.3 m as well as 1.61 Gbps/20 m, indicating its superior long-distance communication ability [4]. Whereas the research on a series micro-LED array-based VLC has only focused on blue light, no relevant study has considered the utilization of series micro-LED arrays with different emission wavelengths to effectively improve VLC throughput performance by using wavelength division multiplexing (WDM).
In this paper, we fabricated multicolor series connection micro-LED arrays with emission wavelengths of violet, blue, green, and yellow and investigated their optoelectronic properties and communication performances. All the micro-LED arrays in series exhibit a multiple increase in LOP, whereas mostly keeping a slightly reduced modulation bandwidth, thus, achieving a higher signal-to-noise ratio (SNR). The micro-LED arrays with emission wavelengths of 400, 451, 509, and 556 nm achieved data rates of 5.71, 4.86, 4.39, and 0.82 Gbps, respectively. The proof-of-concept WDM system was demonstrated with an aggregate data rate of 15.78 Gbps under a transmission distance of 13 m using bit/power loading OFDM, which was the best data rate-distance product performance for the LED-based VLC to the best of our knowledge as shown in Table 1 [12–20]. Summary of LED-Based WDM-OWC Systems with the Aggregate Data Rate over 10 Gbps in Recent Years μLEDs: micro-LEDs. Real WDM: dichroic mirrors and optical color filters are utilized to separate WDM channels. VRGBYC: violet, red, green, blue, yellow, and cyan. Proof-of-concept: an ideal WDM system to directly accumulate the data rates measured individually for each color channel.LED Type System Type Aggregate Distance Data Rate × Performance of the Reference LED (R) + μLEDs (GB) Real WDM 11.28 Gbps 1.5-m free space 16.92 Gbps m 7.09 Gbps m (B) [ 3.84 Gbps m (G) 6 Gbps m (R) LEDs (RGBYC Proof of concept 15.17 Gbps 1.2-m underwater 18.2 Gbps m 3.76 Gbps m (B) [ 3.74 Gbps m (C) 3.72 Gbps m (G) LEDs (RGBY) Proof-of-concept 19.66 Gbps 1.6-m free space 31.46 Gbps m 8.35 Gbps m (B) [ Real WDM 16.92 Gbps 1.6-m free space 27.07 Gbps m 8.42 Gbps m (G) 8.21 Gbps m (R) LEDs (eight color) Proof of concept 20.09 Gbps 1.2-m underwater 24.11 Gbps m 3.46 Gbps m (B) [ 3.29 Gbps m (G) 3.79 Gbps m (R) Mini-LEDs (tricolor) Proof of concept 16.6 Gbps 2-m free space 33.2 Gbps m 14 Gbps m (B) [ 14 Gbps m (C) 5.2 Gbps m (G) LEDs (eight color) Proof of concept 24.25 Gbps 1.2-m free space 29.1 Gbps m 3.98 Gbps m (B) [ 3.91 Gbps m (G) 4.15 Gbps m (R) LEDs (tricolor) + μLEDs (five color) Proof of concept 25.2 Gbps 0.25-m free space 6.3 Gbps m 1.21 Gbps m (V) [ 1.09 Gbps m (B) 1.04 Gbps m (G) μLEDs (UVA + UVB + UVC) Real WDM 10.32 Gbps 0.5-m free space 5.16 Gbps m 1.57 Gbps m (UVA) [ Proof of concept 11.48 Gbps 0.5-m free space 5.74 Gbps m 1.51 Gbps m (UVB) 2.08 Gbps m (UVC) LEDs (RGB) Proof of concept 3.1 Gbps 5-m fiber 15.5 Gbps m 60 Gbps m (B) [ 2 Gbps 100-m fiber 200 Gbps m 60 Gbps m (G) 80 Gbps m (R) μLEDs (VBGY) Proof of concept 15.78 Gbps 13-m free space 205.1 Gbps m 74.23 Gbps m (V) This paper 63.18 Gbps m (B) 57.07 Gbps m (G)
Sign up for Photonics Research TOC. Get the latest issue of Photonics Research delivered right to you!Sign up now
2. SERIES CONNECTION MICRO-LED ARRAYS
A. Design and Fabrication
The fabrication process of series connection micro-LED arrays with different emission wavelengths was started by deposition of 40-nm indium tin oxide (ITO) on the epitaxial layers grown on the -plane sapphire substrate as the current spreading layer. The ITO layer was then patterned by photolithography and wet etching, and GaN was etched to the layer using inductively coupled plasma (ICP) etching. The layer was then patterned with a wider mesa and deeply etched to the sapphire substrate by ICP. Then the epitaxial wafer was annealed under a nitrogen atmosphere at 550°C for 15 min to form ohmic contacts, and the 300-nm layer was grown by plasma-enhanced chemical vapor deposition as an insulating layer. The and electrodes of each pixel were formed by magnetron sputtering deposition of Ti/Al/Ti/Au (50 nm/200 nm/50 nm/250 nm), and the and electrodes of different pixels were interconnected to form a series array. The series connection micro-LED arrays prepared in this paper consist of , , , and layouts of square micro-LED pixels, and the size of each pixel can be designed to be 20 or 40 μm. The center-to-center spacings between 20-μm pixels as well as 40-μm pixels are 90 and 110 μm, respectively. In the series configuration, the pad of the preceding pixel is sequentially connected to the pad of the following pixel by metallic wires with the widths of 20 and 40 μm for the 20- and 40-μm pixels, respectively. The schematic and scanning electron microscopy image of the prepared micro-LED array are shown in Figs. 1(a) and 1(b). Optical micrograph images of the series connection micro-LED arrays with violet, blue, green, and yellow emission wavelengths are shown in Fig. 1(c).
Figure 1.(a) Schematic of GaN-based series connection micro-LED array. (b) Plan-view SEM image of the 20-μm blue GaN-based series connection micro-LED array. (c) Plan-view optical micrograph images of the series connection micro-LED arrays.
B. Optical and Electrical Characteristics
The optical and electrical characteristics of the series connection micro-LED arrays were measured after the chips have been packaged on printed circuit boards. The curves of current versus voltage of the series arrays (B1, B4, B6, and B9 corresponding to 1, 4, 6, and 9 blue pixels, respectively) with different emission wavelengths described above are shown in Fig. 2. As can be seen from Fig. 2, the driving voltage of micro-LED arrays with the same number of pixels at longer wavelength tends to be higher at the same bias current, which may be due to the difference in epitaxial materials and structures. Taking the blue micro-LED array as an example, as the number of pixels increases, the forward bias voltages (3.2, 13.1, 19.4, and 32.0 V) of the series arrays corresponding to a bias current of 1 mA will increase accordingly (1, 4, 6, and 9 pixels). Additionally, the differential resistance of the device under the same bias current will increase as the number of pixels increases. The impact of the RC effect on the modulation bandwidth of the series connection micro-LED array is under investigation due to the discovery of the negative capacitance effect under the forward current.
Figure 2.Current versus voltage curves for (a) 20-μm violet, (b) 20-μm blue, (c) 20-μm green, and (d) 40-μm yellow micro-LED arrays with 1, 4, 6, and 9 pixels in series, respectively.
Series connection is an effective way to boost the LOP of the micro-LED but at the cost of the increased driving voltage. The LOPs of the series connection micro-LED arrays under different current densities are shown in Fig. 3, which are measured by using a lens to focus the light emitted by the array into an optical power meter (Thorlabs PM100D). The LOPs for the 20-μm blue series micro-LED arrays with 1, 4, 6, and 9 pixels under the current density of are 1.09, 4.35, 6.45, and 9.52 mW, respectively, which roughly increase linearly with the increasing number of pixels. The lower LOPs obtained by the yellow and green micro-LED arrays depend on their own lower IQE.
Figure 3.Light output power versus current density curves for (a) 20-μm violet, (b) 20-μm blue, (c) 20-μm green, and (d) 40-μm yellow micro-LED arrays with 1, 4, 6, and 9 pixels in series, respectively.
To confirm the wavelength separation of the micro-LED arrays, which are expected to realize WDM communication, we measured the electroluminescence (EL) spectra of the devices under different current densities as shown in Figs. 4(a)–4(d). The EL spectra of the devices under the communication test conditions are shown in Fig. 4(e). Among them, the peak wavelengths of violet, blue, and green light are 400, 451, and 509 nm, respectively. Due to the existence of stress-induced polarization fields and band-filling effects in the multiple quantum wells with high indium component, the emission wavelength of yellow light has a significant blueshift with the increase in current density [21] as shown in Fig. 4(d), and, thus, the peak wavelength of yellow light is selected to be 556 nm at a lower current density of according to the spectrum separation.
Figure 4.Emission spectra of the (a) 20-μm
3. MODULATION BANDWIDTH
Modulation bandwidth of the light emitter is one of the critical parameters in VLC applications. In this paper, a vector network analyzer (VNA, PicoVNA 106) was adopted to measure the frequency responses of the micro-LEDs. The alternating current (AC) frequency sweep signal from the VNA plus a direct current (DC) signal through a bias-tee (Pasternack PE1615, 0.01–6 GHz) was loaded to the micro-LED to measure the frequency response. The used detector here was a Si avalanche photodiode (APD, Hamamatsu C5658) with a 1-GHz bandwidth. According to Shannon–Hartley theorem [22], the achieved maximum communication data rate will be determined by the channel bandwidth , the average received signal power , and the average noise power . To maximize the performance of long-distance communication systems, we evaluated the achievable bandwidths and LOPs of blue micro-LED arrays with different pixel sizes under their own stable operating current densities as an example. As can be seen from Table 2, a pixel size of 20 μm has the potential to achieve a balance between LOP and bandwidth, so later in the communication performance test, the pixel sizes of blue, green, and violet micro-LEDs were selected as 20 μm; whereas the pixel size of yellow micro-LED was selected as 40 μm because the yellow light was obtained at a low current density as mentioned above and 40-μm pixel exhibited a better performance to balance LOP and bandwidth under low operating current density comparing to that of a 20-μm pixel. In addition, a yellow micro-LED array was selected in this paper to ensure better working stability of the device and communication system. Performances of Blue Micro-LED Arrays with Different Pixel Sizes and Different Operating Current DensitiesPixel Size Stable Operating Current Density/LOP Achieved LOP·Bandwidth 40 μm ( 84.2 MHz 1160 mW MHz 30 μm ( 122.4 MHz 1468 mW MHz 20 μm ( 214.4 MHz 2041 mW MHz 10 μm ( 360.7 MHz 957.6 mW MHz
In addition, operating at the same injection current density, the bandwidths of the GBYV micro-LED devices with different pixel numbers were extracted as depicted in Fig. 5(a). The bandwidths of the micro-LED arrays mostly present similar slight downward trends as the pixels number increases. The series-biased micro-LED array could maintain high modulation bandwidth, whereas improving the LOP; thus, it is more beneficial for VLC applications, especially for a long-distance scenario. The 20-μm 9-pixel violet array, 20-μm 9-pixel blue array, 20-μm 9-pixel green array, and 40-μm 4-pixel yellow array were selected to characterize the long-distance VLC performance later. The frequency responses of the G9, B9, Y4, and V9 micro-LED devices under their operating current densities for VLC are shown in Fig. 5(b), and their extracted bandwidths are 348.1, 214.4, 93.9, and 421.8 MHz, respectively. Therefore, the G9, B9, and V9 micro-LED arrays are more promising transmitters of long-distance VLC because of their both high LOPs and high bandwidths. Moreover, the microwave reflectance (S11) parameters of blue micro-LED arrays with different numbers of pixels in series under the current density of were measured and plotted on the Smith chart from 10 MHz to 1 GHz as shown in Fig. 5(c). The S11 traces of the series micro-LED arrays plotted on the Smith chart will be closer to the right side open circuit point and further away from the center impedance matching point as the number of pixels increases because the differential resistance of the series array also increases with the increase in pixel numbers. This result is similar to the reported S11 parameters in series VCSEL arrays [23]. We further extracted the impedance magnitude corresponding to the S11 parameter as shown in Fig. 5(d). Additionally, it can be seen that an increase in the number of series pixels leads to a more serious impedance mismatch problem when the frequency is less than 400 MHz. The stronger signal reflection caused by the mismatch between the micro-LED impedance and the output impedance (50 Ω) in the system may also be one of the reasons for the decrease in bandwidth after multiple pixels are connected in series. In future work, it may be possible to verify whether the bandwidth of the series connection micro-LED array is affected by impedance mismatch by converting the output impedance of equipment to better match the micro-LED. Further optimization of the series connection micro-LED design and packaging is expected to improve device bandwidth as well as communication performance.
Figure 5.(a) The modulation bandwidths versus pixel numbers of the GBYV micro-LEDs under the same injection current density. (b) The frequency responses of the series-biased GBYV micro-LED arrays under the specific current density for VLC measurement. (c) The microwave reflectance (S11) parameters of blue micro-LED arrays with different numbers of pixels in series under the current density of
4. VLC MEASUREMENT
A. Experiment Setup
The fabricated series connection micro-LED arrays with larger LOPs are expected to achieve more promising VLC performances at a long distance compared to that of the single-pixel device. In this paper, a proof-of-concept WDM system with a 13-m free-space link was established to experimentally test the long-distance data transmission characteristics of the multicolor series connection micro-LED arrays as shown in Fig. 6. Using a homemade program in MATLAB, the digital OFDM data with a bit and power loading algorithm was generated and loaded into the arbitrary waveform generator (AWG, Tektronix AWG710B, 4.2 GSa/s), which plays a role of the high-speed and high-precision digital-to-analog converter. The output port of the AWG was connected to an electrical amplifier (EA, Mini-Circuits ZHL-2-12+) with a typical gain of 26 dB. Through a bias-tee (Pasternack PE1615, 0.01–6 GHz), the bipolar waveform from EA was biased by a DC signal from a DC source (Yokogawa GS610) to yield the positive signal, which was a must for intensity modulation in optical communication. The unipolar OFDM signal was fed into the series-biased micro-LED array chip to emit modulated light, which was collimated by an aspheric condenser lens (Thorlabs ACL5040U-A, Ø50 mm, , ). Via a mirror, the transmission distance in indoor free space was extended to 13 m. In addition, the reflected light beam was collected and focused onto an APD (Hamamatsu C5658, 1 GHz) or a positive–intrinsic–negative (PIN) photodetector (Femto HSPR-X-I-1G4-SI, 1.4 GHz) using a Fresnel lens with a 10-cm diameter. Considering the joint influences of modulation bandwidth and LOP on transmission performance, the high-bandwidth PIN photodetector was applied for the VLC measurements of violet, blue, and green chips that can provide relatively large LOP; it was replaced as the 1-GHz APD with high sensitivity for the yellow chip. An oscilloscope (Agilent DSA90604A Infiniium) sampled the output from the photodetector with a sampling rate of 20 GSa/s. At last, the captured data was sent to the laptop and demodulated offline. The electronic equipment used in this paper has high bandwidth and, thus, the bandwidth of the micro-LED chip can be regarded as the limitation for the bandwidth of the entire system.
Figure 6.Schematic of the experimental setup for the multicolor series connection micro-LED arrays-based long-distance VLC system.
B. Modulation Scheme
The VLC system has a decay response in the frequency domain resulting in the nonuniform SNR distribution. Therefore, DC-biased optical OFDM (DCO-OFDM) modulation with the bit and power loading method is used in this paper to allocate more bits on each subcarrier to approach Shannon capacity, and its block diagram is shown in Fig. 6. The binary sequences are mapped into -QAM (quadrature amplitude modulation) symbols after serial-to-parallel conversion, where represents the QAM order and is determined by the frequency-dependent SNR on the subcarrier. For the DCO-OFDM scheme, conjugate symbol data is added in the frames to satisfy Hermitian symmetry to generate the real-value data after inverse fast Fourier transform (IFFT). Before IFFT, zero-padding up-sampling and zero-forcing preequalization are employed to alleviate the spectral sidelobes and to broaden the OFDM signal bandwidth in the frequency domain, respectively. The fixed number of subcarriers that load the QAM symbols is set as 512, and half of the subcarriers are used to load the corresponding conjugate values. Then, the serial discrete-time signal is sent into the AWG after the processes of adding the cyclic prefix and parallel-to-serial conversion. In the demodulation part, the channel estimation with least-squares method is used to suppress channel selective fading response. At last, the opposite processes of OFDM modulation are performed to recover the binary data and calculate the bit error ratio (BER) value.
Before implementing the processes above, the binary phase shift keying symbols with identical subcarrier power ought to be sent first to carry out the SNR estimation and the frequency response of the overall system is obtained. At a target BER, afterward, bit loading method could adaptively allocate graded QAM orders as an initial allocation according to the SNR distribution, which is derived from the error vector magnitude between the transmitted and the received constellations. However, the initial allocation has a considerable current margin, which is the gap between the estimated subcarriers SNR and required SNR for current allocated QAM order. Current margin is used to evaluate the degree of wasted energy. The allocated bits amount will be improved when the current margin is reduced. Hence, the power loading method is adopted to optimize the SNR margin and update the bit allocation by adjusting the power ratio on the subcarriers according to the Levin–Campello algorithm [24]. It is noted that the little variation of power on the subcarriers leads to the equivalent variation of SNR in this paper. Jointly employing the bit and power loading, the spectral efficiency will be improved. Based on the updated bit allocation, the data transmission rate is calculated by [17]
C. Results and Discussion
The VLC performances of the GBYV series connection micro-LED arrays were conducted individually based on the experiment setup and modulation scheme described above. The driving current and modulation depth () are two critical parameters that will significantly affect the transmission performance. When the micro-LED is biased at a very low or a very high DC value, the zero-level clipping or optical power saturation will occur and lead to SNR deterioration. Additionally, a very high DC bias may damage the micro-LED chip owing to the strong heating effect. Through comprehensive considerations of the system data rate and stability, the values of DC bias are set to 24 mA (), 16 mA (), 16 mA (), and 1.6 mA () for 400-, 451-, 509-, and 556-nm micro-LEDs, respectively. In addition, the LOPs of the 20-μm violet and blue micro-LED arrays at the current density corresponding to their communication test conditions (violet is and blue is ) as a function of operating time measured without encapsulation are shown in Fig. 7(a). During the 200-min operation, the LOPs of the blue and violet micro-LED arrays will first increase slightly and then stabilize or decrease slightly with fluctuations of no more than 1.5%, indicating that the series connection micro-LED array can achieve stable operation within a certain long time. The good stability benefits from the uniform current spreading and good heat dissipation of the micro-LED. Further encapsulation of the series connection micro-LED arrays to form modules using the transistor-outline-can package, copper heat sink, and a thermoelectric cooler is required to ensure their long-term operating stability. In addition, a low value of will cause the incomplete utilization of the linear region, and a high value of will cause severe nonlinear distortion. The optimum is corresponding to the highest average SNR as shown in Fig. 7(b), which will be amplified by the amplifier with a typical gain of 26 dB. Additionally, the values of from the AWG are set to 0.8, 0.65, 2, and 0.325 V for 400-, 451-, 509-, and 556-nm micro-LEDs, respectively. In practice, an appropriate value of the signal modulation bandwidth will lead to a highest data transmission rate, and it is affected by both the received optical power and the system frequency response. The signal modulation bandwidths of 400-, 451-, and 509-nm micro-LED arrays are set to larger values of 1200, 1200, and 1050 MHz, respectively, benefiting from their larger received optical powers and bandwidths. For the opposite reason, the signal bandwidth of the 556-nm micro-LED array is fixed at a smaller value of 500 MHz. In the signal band, the distributions of SNR, bit number, and power ratio on the subcarriers are obtained at the target BER of as shown in Fig. 8. It can be seen that the SNR at low frequency of the system is relatively low, mainly due to the low responses of the bias-tee and amplifier at low frequency. The initial power allocations on the subcarriers are identical, and thereby the value of the power ratio is set as 1 initially. Then, after carrying out the power loading, the power ratios on the subcarriers fluctuate around 1 as shown in Fig. 8, whereas the total power is constant.
Figure 7.(a) Light output powers of the 20-μm
Figure 8.SNR distributions, bit allocations, and power ratios versus frequency for (a) 400-nm, (b) 451-nm, (c) 50-nm, and (d) 556-nm channels.
The average numbers of bits per symbol are 4.7578, 4.0493, 4.1809, and 1.6368 for 400-, 451-, 509-, and 556-nm micro-LED arrays, resulting in the achievable data rates of 5.71, 4.86, 4.39, and 0.82 Gbps, respectively. Therefore, corresponding to an ideal WDM implementation without considering the channel cross talk, an aggregate data rate of 15.78 Gbps is achieved, which would be indeed decreased in real scenarios. The power spectra with the use of pre-equalization for the GBYV chips are shown in Fig. 9. Certainly, a decrease in the SNR and data rate for each channel will be observed in a real WDM demonstration with dichroic mirrors and optical filters due to the channel cross talk induced by the spectral overlap. In this paper, the violet, blue, green, and yellow micro-LED channels can be separated by using 420-, 490-, and 550-nm long pass dichroic mirrors, respectively. Taking the blue micro-LED channel as an example, the performance loss of the channel was evaluated by adding a 420 nm long pass filter at the receiver. The data rate of the blue micro-LED channel would be reduced by 7.4% from 4.86 to 4.5 Gbps under the same transmission distance. In addition, for green and yellow micro-LED channels, the data rate loss is more severe due to more spectral overlap. Figure 10(a) depicts the constellation plots for the 400-nm channel including the 4, 8, 16, 32, 64, and 128 QAMs. To verify the superiority of series connection micro-LED array in the long-distance VLC application, the SNR distributions of the violet single-pixel and series connection devices under the same injection current density of before preequalization are acquired for direct comparison as shown in Fig. 10(b). It is obvious that the series connection micro-LED array exhibits a high SNR within the signal bandwidth and provides an average SNR of 16.86 dB, which is improved by 6.26 dB compared with that of the 20-μm single-pixel device. Although the violet devices with different pixel numbers have similar bandwidths, the series connection device with higher LOP could offer higher SNR and is more suitable for a long-distance VLC.
Figure 9.Power spectra for (a) 400-nm, (b) 451-nm, (c) 509-nm, and (d) 556-nm channels.
Figure 10.(a) Constellation plots for the 400-nm channel including the 4, 8, 16, 32, 64, and 128 QAMs. (b) SNR distributions of the 20-μm violet single-pixel and series connection devices under the current density of
5. CONCLUSION
In this paper, we prepared the multicolor series connection micro-LED array devices and experimentally investigated their superiority in long-distance VLC applications. The designed series connection micro-LED array with a pixel size of 20 μm exhibited the light output power of multiple milliwatts, whereas mostly keeping a slightly reduced modulation bandwidth compared with the single-pixel device. The decrease in optical bandwidth may be caused by the mismatch between the impedance of the micro-LED operating at high frequency as well as high current and the system output impedance, which can be improved by impedance matching methods, such as device structure design and package design. In addition, we have prepared yellow micro-LED arrays and applied them to long-distance communication for the first time. In addition, then, we use the G9, B9, Y4, and V9 series-biased micro-LED array devices as the transmitters of the VLC system with a 13-m free-space link. Based on the OFDM modulation with the bit and power loading scheme, the G9, B9, Y4, and V9 devices could provide the data rates of 4.86, 4.39, 0.82, and 5.71 Gbps, respectively. In addition, we investigate the effect of single-pixel and multipixel series-biased micro-LED devices with the same pixel size on the SNR distribution. It is verified that the series-biased micro-LED device could offer a higher SNR within the same signal bandwidth. Thus, the series-biased micro-LED device will certainly bring a higher data transmission rate in such a long distance. The total data rate of 15.78 Gbps was achieved for the proof-of-concept WDM system, and it had the best data rate-distance product performance for the LED-based VLC to the best of our knowledge. In the future, the GBYC series connection micro-LED devices are expected to be adopted in a real long-distance WDM demonstration.
References
[1] M. Katz, I. Ahmed. Opportunities and challenges for visible light communications in 6G. 2nd 6G Wireless Summit (6G SUMMIT), 1-5(2020).
[2] S. Zhu, X. Chen, X. Liu, G. Zhang, P. Tian. Recent progress in and perspectives of underwater wireless optical communication. Prog. Quantum Electron., 73, 100274(2020).
[3] A. Rashidi, M. Monavarian, A. Aragon, A. Rishinaramangalam, D. Feezell. Nonpolar
[4] E. Xie, R. Bian, X. He, M. S. Islim, C. Chen, J. J. D. McKendry, E. Gu, H. Haas, M. D. Dawson. Over 10 Gbps VLC for long-distance applications using a GaN-based series-biased micro-LED array. IEEE Photon. Technol. Lett., 32, 499-502(2020).
[5] P. Tian, J. J. McKendry, Z. Gong, B. Guilhabert, I. M. Watson, E. Gu, Z. Chen, G. Zhang, M. D. Dawson. Size-dependent efficiency and efficiency droop of blue InGaN micro-light emitting diodes. Appl. Phys. Lett., 101, 231110(2012).
[6] Z. Yuan, Y. Li, X. Lu, Z. Wang, P. Qiu, X. Cui, P. Tian, Q. Wang, G. Zhang. Investigation of modulation bandwidth of InGaN green micro-LEDs by varying quantum barrier thickness. IEEE Trans. Electron Devices(2022).
[7] Y.-H. Chang, Y.-M. Huang, F.-J. Liou, C.-W. Chow, Y. Liu, H.-C. Kuo, C.-H. Yeh, W. H. Gunawan, T.-Y. Hung, Y.-H. Jian. 2.805 Gbit/s high-bandwidth phosphor white light visible light communication utilizing an InGaN/GaN semipolar blue micro-LED. Opt. Express, 30, 16938-16946(2022).
[8] Y.-H. Chang, Y.-M. Huang, W. H. Gunawan, G.-H. Chang, F.-J. Liou, C.-W. Chow, H.-C. Kuo, Y. Liu, C.-H. Yeh. 4.343-Gbit/s green semipolar (20-21)
[9] M. Monavarian, A. Rashidi, D. Feezell. A decade of nonpolar and semipolar III-nitrides: a review of successes and challenges. Phys. Status Solidi A, 216, 1800628(2019).
[10] H.-Y. Lan, I.-C. Tseng, Y.-H. Lin, G.-R. Lin, D.-W. Huang, C.-H. Wu. High-speed integrated micro-LED array for visible light communication. Opt. Lett., 45, 2203-2206(2020).
[11] G.-R. Lin, H.-C. Kuo, C.-H. Cheng, Y.-C. Wu, Y.-M. Huang, F.-J. Liou, Y.-C. Lee. Ultrafast 22 green micro-LED array for optical wireless communication beyond 5 Gbit/s. Photon. Res., 9, 2077-2087(2021).
[12] H. Chun, S. Rajbhandari, G. Faulkner, D. Tsonev, E. Xie, J. J. D. McKendry, E. Gu, M. D. Dawson, D. C. O’Brien, H. Haas. LED based wavelength division multiplexed 10 Gb/s visible light communications. J. Lightwave Technol., 34, 3047-3052(2016).
[13] Y. Zhou, X. Zhu, F. Hu, J. Shi, F. Wang, P. Zou, J. Liu, F. Jiang, N. Chi. Common-anode LED on a Si substrate for beyond 15 Gbit/s underwater visible light communication. Photon. Res., 7, 1019-1029(2019).
[14] R. Bian, I. Tavakkolnia, H. Haas. 15.73 Gb/s visible light communication with off-the-shelf LEDs. J. Lightwave Technol., 37, 2418-2424(2019).
[15] F. Hu, G. Li, P. Zou, J. Hu, S. Chen, Q. Liu, J. Zhang, F. Jiang, S. Wang, N. Chi. 20.09-Gbit/s underwater WDM-VLC transmission based on a single Si/GaAs-substrate multichromatic LED array chip. Optical Fiber Communications Conference and Exhibition (OFC), M3I.4(2020).
[16] X. Liu, Z. Wei, M. Li, L. Wang, Z. Liu, C. Yu, L. Wang, Y. Luo, H. Fu. Experimental investigation of 16.6 Gbps SDM-WDM visible light communication based on a neural network receiver and tricolor mini-LEDs. Opt. Lett., 46, 2888-2891(2021).
[17] F. Hu, S. Chen, G. Li, P. Zou, J. Zhang, J. Hu, J. Zhang, Z. He, S. Yu, F. Jiang, N. Chi. Si-substrate LEDs with multiple superlattice interlayers for beyond 24 Gbps visible light communication. Photon. Res., 9, 1581-1591(2021).
[18] P. Qiu, S. Zhu, Z. Jin, X. Zhou, X. Cui, P. Tian. Beyond 25 Gbps optical wireles communication using wavelength division multiplexed LEDs and micro-LEDs. Opt. Lett., 47, 317-320(2022).
[19] D. M. Maclure, J. J. McKendry, M. S. Islim, E. Xie, C. Chen, X. Sun, X. Liang, X. Huang, H. Abumarshoud, J. Herrnsdorf, E. Gu, H. Haas, M. D. Dawson. 10 Gbps wavelength division multiplexing using UV-A, UV-B, and UV-C micro-LEDs. Photon. Res., 10, 516-523(2022).
[20] J. Shi, Y. Zhou, N. Chi, L. Xiong, J. Luo. Gigabit LED-based visible light transparent transmission from free-space to a 100-m ultra-large effective area pure silica fiber. Microw. Opt. Technol. Lett., 60, 13-18(2018).
[21] C. Du, Z. Ma, J. Zhou, T. Lu, Y. Jiang, P. Zuo, H. Jia, H. Chen. Enhancing the quantum efficiency of InGaN yellow-green light-emitting diodes by growth interruption. Appl. Phys. Lett., 105, 071108(2014).
[22] H. Chun, S. Rajbhandari, G. Faulkner, E. Xie, J. J. D. McKendry, E. Gu, M. D. Dawson, D. O’Brien. Optimum device and modulation scheme selection for optical wireless communications. J. Lightwave Technol., 39, 2281-2287(2021).
[23] J.-L. Yen, X.-N. Chen, K.-L. Chi, J. Chen, J.-W. Shi. 850 nm vertical-cavity surface-emitting laser arrays with enhanced high-speed transmission performance over a standard multimode fiber. J. Lightwave Technol., 35, 3242-3249(2017).
[24] F. Hu, S. Chen, Y. Zhang, G. Li, P. Zou, J. Zhang, C. Shen, X. Zhang, J. Hu, J. Zhang, Z. He, S. Yu, F. Jiang, N. Chi. High-speed visible light communication systems based on Si-substrate LEDs with multiple superlattice interlayers. PhotoniX, 2, 16(2021).
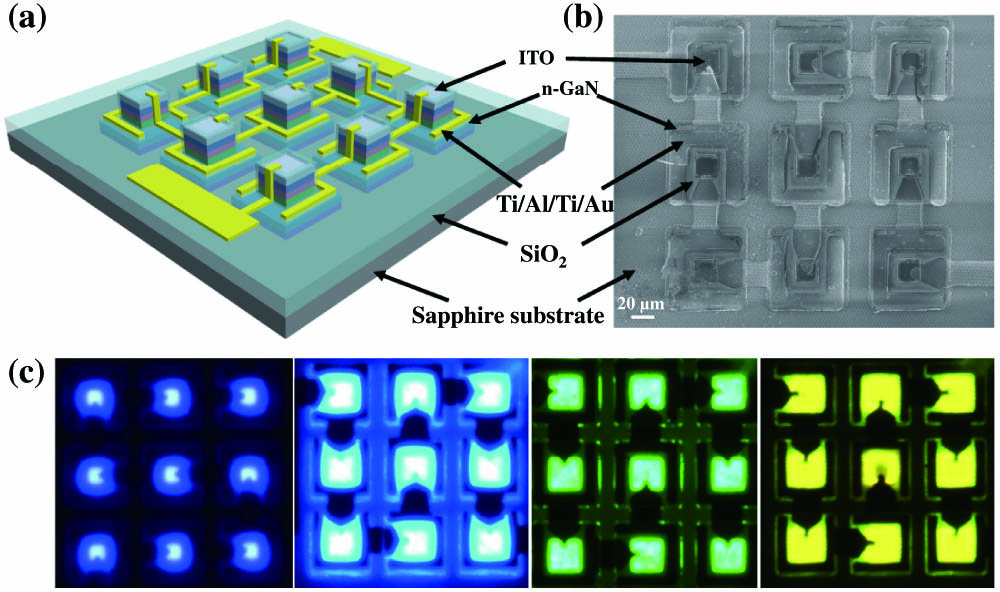
Set citation alerts for the article
Please enter your email address