
- Photonics Research
- Vol. 11, Issue 10, 1635 (2023)
Abstract
1. INTRODUCTION
Nowadays, the rare spectrum resource has become one of the major factors limiting the development of wireless communication, not only for B5G and 6G networks, but also for the new generation satellite communications [1]. In-band full-duplex (IBFD) technology can realize simultaneously transmitting and receiving radio frequency (RF) signals at the same frequency band, which significantly increases spectrum efficiency and doubles the data transmission rate [2–4]. However, during IBFD communication, the high-power signal sent from the transmitting antenna will interfere with the low-power signal of interest (SOI), which is also received by the receiving antenna. It is called RF self-interference, and cannot be filtered out by a notch filter due to the spectrum overlap between the SOI and the interference. Therefore, RF self-interference cancellation (SIC) is essential for the application of IBFD technology.
RF SIC technology includes spatial cancellation, analog domain cancellation, and digital domain cancellation [5–8]. Among them, the analog domain cancellation is a very important stage to suppress RF self-interference to enough low-power levels to ensure that the analog to digital conversion (ADC) unit avoids being saturated and works within the dynamic range. The electronic methods for analog domain RF SIC are generally limited by the bandwidth as well as the amplitude and phase tuning precision of RF devices, and it is difficult to obtain high cancellation depth over a wide RF band.
Compared with electronic methods, photonic SIC (PSIC) has the advantages of wide bandwidth, high amplitude and delay tuning precision, and immunity to electromagnetic interference. In recent years, many kinds of PSIC schemes have been proposed with demonstration of the above advantages [9]. The dual Mach–Zehnder modulator (MZM)-based PSIC system was first reported to suppress RF self-interference, which was biased at the orthogonal points of the positive slope and negative slope [10]. Since then, PSIC schemes have been presented successively by using a dual-drive MZM (DD-MZM) [11], dual-parallel MZM (DP-MZM) [12], and DP polarization modulator (DP-PolM) [13], where the phase inversion between the interference path and reference path was completed by direct current (DC) bias control. Phase inversion can also be realized in the optical domain by phase modulation and sideband filtering [14] and cross-gain modulation of a semiconductor optical amplifier [15], or in the electronic domain by using Balun [16] and a balanced photodetector (PD) [17,18]. For a realistic scenario, multipath PSIC schemes should be considered to eliminate interference signals caused by reflection, scattering, and diffraction. Multipath PSIC systems were constructed through a single-mode to multimode (SM-MM) combiner [19,20], dispersive element [21], phase modulation and wavelength division multiplexing [22], and fiber Bragg grating (FBG)-based delay line [23,24]. Recently, RF signals over fiber transmission [25], frequency downconversion [26,27], and image-reject mixing [28] were also studied when achieving RF SIC at the same time. The above-mentioned schemes have made impressive contributions to the progress of IBFD communication. However, they are all based on discrete optoelectronic devices, which may suffer from bulky volume and high power consumption, and cannot satisfy the miniaturization requirement of mobile terminals, unmanned aerial vehicles (UAV), and small satellite payloads.
Sign up for Photonics Research TOC. Get the latest issue of Photonics Research delivered right to you!Sign up now
The rapid development of photonic integration technology gives impetus to the advance of microwave photonics (MWP) technology [29,30]. Through integration of discrete optoelectronic devices on chip, the size, weight, and power consumption (SWaP) of the MWP system and the coupling loss between devices can be tremendously reduced, and system stability can be improved greatly [31]. The integrated PSIC chip was first proposed and characterized on an indium phosphide (InP) platform [32,33]. It utilized a pair of DC-driven semiconductor optical amplifiers to realize the adjustment of amplitude and phase of the reference signal, with the RF SIC demonstration in Long Term Evolution (LTE) and WiFi bands. Continuous work was implemented to improve the link gain and noise figure by the external modulation and balanced detection [34]. The silicon photonic integrated chip has the benefits of the ability for high laser powers and lower process variation yield, and has been attracting much attention for PSIC in recent years. The silicon photonic integrated MWP filter for amplitude tuning with off-chip delays was primarily investigated for RF SIC [35]. The DP-MZM was designed and fabricated on IMEC’s silicon-on-insulator iSiPP50G platform for the application of RF SIC [36], with the tuning of amplitude and delay time by RF devices. However, the instantaneous bandwidths and operation RF band of the reported integrated PSIC systems are relatively narrow.
In this paper, the first silicon integrated PSIC chip is presented and demonstrated experimentally. Here, the phase modulator (PM), variable optical attenuator (VOA), tunable optical delay line (TODL), wavelength division multiplexer (WDM), optical filter (OF), and PD are fully integrated on one chip to construct an MWP subsystem with the function of regulating the amplitude and time delay of optically carried RF signals to cancel the RF self-interference over a broad band. The scheme has the advantages of a specific out-of-phase property between the right and left sidebands of optically filtered phase modulation signals, and no need for complicated DC bias control as with intensity modulation [10–13]. Through a comprehensive investigation including the function unit and subsystem design, PSIC chip characterization, and optical, electrical, and RF co-package, the integrated PSIC module operates over the broad range of C, X, Ku, and K bands. The IBFD in Ku band of satellite communication is demonstrated with the successful recovery of the SOI. The factors affecting the cancellation depth and maximum interference to SOI ratio of the integrated PSIC system are measured and analyzed in detail. In addition, the performances of the integrated PSIC system including the link gain, noise figure, receiving sensitivity, and spurious free dynamic range (SFDR) are characterized.
2. PSIC SYSTEM ARCHITECTURE AND CHIP DESIGN
The structure of the integrated PSIC system on a silicon photonic platform is shown in Fig. 1. The operation principle of the proposed PSIC system is based on phase modulation and optical sideband filtering to realize the phase conversion between reference and interference signals. The received signals, including the SOI
Figure 1.Structure of the integrated PSIC system and schematic spectra illustrating phase modulation and sideband filtering. PM, phase modulator; VOA, variable optical attenuator; TODL, tunable optical delay line; WDM, wavelength division multiplexer; OF, optical filter; PD, photodetector.
The VOA is composed of a Mach–Zehnder interferometer (MZI) structure with a phase shifter on one arm to implement the output intensity tuning. This MZI is a symmetric structure with equal interference arms of 120 μm. The TODL is formed by cascading two ring resonators with the same perimeter of 125 μm and one phase shifter on each ring waveguide. The ring resonators operate in the anti-resonant state to obtain the time delay with low loss [37]. The delay amount is regulated by adjusting the additional phase on the two rings. The phase modulated interference and reference signals are combined via the WDM and input to the OF. The structure of WDM is an MZI with unequal length arms, which has much lower coupling loss and higher scalability compared with the direction coupler. The arm length difference is 570.5 μm, corresponding to a free spectral range (FSR) of 1 nm. The phase shifter is also designed on one arm to achieve flexible regulation of the multiplexing wavelength. To realize a broad frequency band for SIC, the OF should have a “box-like” filtering response shape to filter out one sideband at low frequency and simultaneously retain the other sideband at high frequency. Here, the ring assisted MZI structure is utilized to implement the function [38]. To regulate the filtering response shape conveniently, the couplers in the OF are MZI structures with adjustable coupling coefficients [39]. The phase shifters are also set on the ring waveguide and the long arm waveguide to realize phase tuning to optimize the filtering response. The sideband filtering of phase modulation signals is completed by the OF as the schematic spectra at point C shows in Fig. 1. The
Silicon photonics leverages the maturity and know-how of silicon complementary metal–oxide semiconductor (CMOS) processing techniques and is a suitable platform for large-scale photonic integrated circuits [40]. The integrated PSIC system was fabricated on a standard 220 nm silicon-on-insulator platform. Figure 2(a) shows the optical microscope image of the PSIC chip with an area of
Figure 2.(a) Optical microscope image of the integrated PSIC chip. (b) Cross-sectional schematic view (not to scale) of the silicon-on-insulator platform. (c) Packaged PSIC module. AGC, array grating coupler; TEC, thermo-electric cooler; FA, fiber array.
3. EXPERIMENTAL RESULTS
A. Test of Function Units in the PSIC Chip
Before the RF SIC measurement, the function units in the PSIC chip including the VOA, TODL, WDM, OF, and PD were characterized first.
1. VOA
The VOA acts as the amplitude tuning unit for the phase modulated RF signal in the optical domain. The phase shifter on one of the arms of MZI is based on the thermo-optic effect. The TiN heater with a thickness of 120 nm and length of 100 μm was measured with a resistance of 580 Ω. The second and fourth waveguide grating couplers, namely, ② and ④ in Fig. 1, are utilized as the input and output optical ports for measurement, respectively. The cross optical output was monitored with an optical power meter (EXFO, FPM-602) when the DC voltage from the multi-channel power (NI, PXIe-4163) was applied on the heater. The relationship between the output optical power and applied DC power is plotted in Fig. 3(a) exhibiting a cosine curve. According to the operation principle of MZI-based VOA, the required DC power to obtain a phase shift of
Figure 3.Measured results of VOA. (a) Variation curve of output optical power with applied DC power. (b) Measured optical spectrum of different applied powers over 2.54 nm bandwidth.
Subsequently, the amplitude tuning response over a certain wavelength range was measured by the optical vector analyzer (OVA, LUNA 5000), and the results are shown in Fig. 3(b). The standard resolution of scanning wavelength is 1.6 pm. The amplitude response is flat over 2.54 nm, about 317.5 GHz, which ensures equal amplitude tuning for a broad band. A maximum amplitude tuning range of 33 dB is realized with the power of about 44.8 mW, which corresponds to a thermal regulated phase difference of
2. TODL
The delay response of the TODL unit on chips was characterized by the OVA. With a single scanning, the OVA can simultaneously measure the complete transfer response of photonic devices including insertion loss and group delay versus wavelength. Figure 4(a) shows the relative intensity response of the two cascaded rings with different driving DC powers (Pr1 and Pr2) on the two heaters. The resonant wavelengths of the two rings align with each other when DC powers are 10.3 mW and 11.4 mW. The measured FSR of the ring is 4.5 nm, which is consistent with the designed ring perimeter. Figures 4(b) and 4(c) show the zoom-in intensity response around 1550 nm and the corresponding time delay response. As can be seen clearly, in case 1,
Figure 4.Measured optical spectrum of TODL. (a) Intensity response under different regulation states. (b) Zoom-in view of (a) around 1550 nm. (c) Group delay response corresponding to different regulation states in (b).
3. WDM
The MZI-based WDM is designed as a combiner for two different optical wavelengths. Figure 5(a) shows the simulation results, where the red curve is the intensity response from input port I1 to output port O1, and the blue curve is from input port I2 to output port O1. To ensure low transmission loss, wavelengths of
Figure 5.Transmission spectra of the WDM. (a) Simulated result. (b) Measured intensity response with different applied powers.
4. OF
The OF implements the function of sideband filtering to realize conversion from phase modulation to intensity modulation and the phase inversion between interference and reference branches. To obtain the box-like filtering response of the ring assisted MZI structure, five phase shifters in the OF as shown in Fig. 1 are designed to tune the coupling coefficients and the additional phase on the ring waveguide and the long arm waveguide. The filtering response can be characterized easily because the PSIC chip has been packaged with fiber array and DC ports. The sixth and seventh waveguide grating couplers, namely, ⑥ and ⑦ in Fig. 1, are utilized as the input and output optical ports for measurement, respectively, which can represent the filtering response from the WDM to the PD due to the symmetric property of the ring assisted MZI structure. Figure 6 shows the measured intensity (blue) and phase (red) response curves of the OF. The out-of-band rejection ratio is 19 dB, the 3 dB bandwidth is 0.45 nm, and the FSR is 1 nm. According to the 3 dB bandwidth and sideband filtering principle, the maximum frequency band of about 56 GHz can be operated. It can be seen from Fig. 6 that the phase response within the passband is linear, and it indicates that the time delay is equal in the operation frequency band.
Figure 6.Measured intensity and phase response of the OF.
5. PD
PD is used for the optical-electrical (O/E) conversion. As marked in Fig. 2, the characterized PD unit is composed of a waveguide grating coupler and a germanium PD. We tested the PD unit using a vector network analyzer (VNA, Keysight N5247B) and a commercial high-bandwidth intensity modulator (MZM, EO-Space 40 GHz). The RF signal from VNA is modulated on the optical carrier of 1550 nm via the intensity modulator. Here, the MZM operates at the orthogonal point by using a bias point controller. The optically carried RF signal is directly coupled into the on-chip PD through the waveguide grating coupler. The RF probe (GSG, 40 GHz) detects the output RF signal from the PD biased with reverse DC voltage. A bias-tee is used to connect the RF probe and the cable to the input port of the VNA, by which reverse DC voltage can be applied to the PD via the probe, and the RF signal can transmit to the VNA. The measured O/E conversion response of the PD is shown in Fig. 7 with reverse DC voltage of
Figure 7.Measured O/E conversion response of the PD.
B. RF SIC and IBFD Communication Characterization
The experiment setup as shown in Fig. 8 was established to investigate the feasibility of the PSIC module for IBFD communication.
Figure 8.Experiment setup to demonstrate the feasibility of the PSIC module for IBFD communication.
Figure 9.Optical spectrum of phase-modulated signals by sideband filtering.
1. Operation Bandwidth and Cancellation Depth of PSIC System
The operation bandwidth and cancellation depth of the PSIC system were measured with the scheme shown in the blue dotted line in Fig. 8. The VNA (Keysight N5247B) was used to measure the
Figure 10.
The operation frequencies covering C, X, Ku, and K bands are selected to characterize the cancellation performance at 5 GHz, 8 GHz, 10 GHz, 12.4 GHz, 14.2 GHz, 16 GHz, 18 GHz, 20 GHz, and 25 GHz. Over 1 GHz bandwidth at each central frequency, the VOA and TODL in the reference path are adjusted to make the reference signal and interference signal equal in amplitude and opposite in phase, and then the cancellation depth is characterized. The measured results are shown as the blue curve in Fig. 11. The red line marks the position of the 20 dB cancellation depth, and the orange area is the operation bandwidth for the cancellation depth over 20 dB. It can be seen from Fig. 11 that a cancellation depth of more than 20 dB is achieved with the narrowest bandwidth of 140 MHz for all the selected operation frequencies. A maximum bandwidth of 630 MHz is obtained at a center frequency of 10 GHz.
Figure 11.Cancellation depth characterization in C, X, Ku, and K bands.
The cancellation depth that can be achieved within a certain bandwidth is determined by the amplitude deviation and phase deviation between interference and reference branches. Central frequencies of 12.4 GHz and 14.2 GHz in Ku band satellite communication were selected for further analysis of the factors affecting cancellation performance. Figures 12(a) and 12(b) show the amplitude deviation (blue curve) and phase deviation (red curve) between interference and reference branches measured by VNA over 1 GHz bandwidth at central frequencies of 12.4 GHz and 14.2 GHz, respectively. The orange areas in Figs. 12(a) and 12(b) mark the bandwidth with the cancellation depth over 20 dB, indicating that the maximum phase deviation is 5.7°, and the maximum amplitude deviation is 0.82 dB. These are consistent with the theorical analysis [9].
Figure 12.Measured amplitude and phase differences between reference and interference branches. (a)
2. Recovery of SOI
An experimental verification for the full-duplex satellite communication of Ku band by using the integrated PSIC module was carried out. Additive white Gaussian noise (AWGN) from SG1 with a bandwidth of 100 MHz and power of 18 dBm was utilized as the interference signal. The 16-quadrature amplitude modulation (16QAM) signal with a bandwidth of 50 Msps from SG2 was used as the SOI. After transmitting through the PSIC system, the output signals were measured by an electric spectrum analyzer (ESA, Keysight N9030B). Figures 13(a) and 13(b) show the measured spectra with and without cancellation and the corresponding constellation diagram of recovered SOI. As shown in Fig. 13(a), for a central frequency of 12.4 GHz, the gray curve shows the spectrum without interference cancellation; the SOI is buried by the strong interference signal, and the constellation diagram is cluttered with an error vector magnitude (EVM) of 23.06% rms. After the interference is suppressed, the SOI is recovered as shown in the pink curve with an EVM of 9.46% rms. According to the international 3GPP standard [42], the maximum EVM allowed by 16QAM signal is 12.5% rms. Therefore, the SOI is recovered successfully by the integrated PSIC module. When SG2 is turned off, the red curve shows the spectrum of a residual interference signal, exhibiting a cancellation depth of 24.9 dB. Similarly, as shown in Fig. 13(b), for a center frequency of 14.2 GHz, a cancellation depth of 26.6 dB is achieved with a bandwidth of 100 MHz, and the EVM of the recovered SOI is 8.46% rms.
Figure 13.Output RF spectra in bandwidth of 100 MHz with and without cancellation and constellation diagram of SOI in bandwidth of 50 Msps. (a)
3. Maximum Interference to SOI Ratio
To characterize the SOI recovery capability of the PSIC system, the maximum interference to SOI ratio
First, the central frequency was set at 12.4 GHz, and power and bandwidth of SOI were
Figure 14.Measured EVM of the recovered SOI with different powers of interference signal. (a)
C. MWP System Performance Characterization
1. Gain and Noise Figure
The gain (
2. Receiving Sensitivity
Receiving sensitivity, namely, the minimum signal power that the system can receive for a certain EVM requirement, is utilized to characterize the performance of the PSIC system. At a central frequency of 12.4 GHz, the SOI of 16QAM with a bandwidth of 50 Msps was generated from SG2 and then passed through the PSIC system. The SOI power was increased gradually, and the corresponding EVM values were measured, with the results shown in Fig. 15(a). When the EVM reaches 12.5% rms, the receiving sensitivity of the system is
Figure 15.Measured EVM of SOI after PSIC system with different input powers. (a)
3. Spurious Free Dynamic Range
The SFDR is an important parameter of an MWP system influenced by the gain, noise figure, and nonlinear intermodulation. The two-tone test method [43] was applied to measure the SFDR of the established PSIC system. First, the interference signal was not considered with SG1 being turned off. The SFDR of the PSIC system was measured by using a two-tone signal from SG2 with an interval of 10 MHz loaded on
Figure 16.Measured SFDR of the PSIC system without interference and with cancellation. (a)
SG1 is turned on and the interference signal of 18 dBm is input to the integrated PSIC system and then cancelled by the reference signal. The noise floor of the system is affected by the residual interference signal. However, because the interference signal is suppressed much lower than the SOI, the powers of the fundamental and third-order intermodulation signals are consistent with the results when the interference signal is off. The results are also presented in Fig. 16. It can be seen that the noise floors are
4. CONCLUSION AND DISCUSSION
In this paper, a silicon-based PSIC on-chip system is presented for the first time with a comprehensive investigation including design, measurement, and characterization. The performance comparison of the reported integrated PSIC system is illustrated in Table 1 [32,34–36]. By virtue of phase modulation and optical sideband filtering on chip, the PSIC system is compact and can operate covering C, X, Ku, and K bands. A cancellation depth of greater than 20 dB is achieved with the narrowest bandwidth of 140 MHz. A maximum bandwidth of 630 MHz is obtained at a center frequency of 10 GHz. For potential Ku band satellite communication, the prototype full-duplex communication is carried out by using the integrated PSIC module. Cancellation depths of 24.9 dB and 26.6 dB are obtained at central frequencies of 12.4 GHz and 14.2 GHz with a bandwidth of 100 MHz, respectively. The factors affecting the cancellation depth and the maximum interference to SOI ratio are measured and analyzed in detail. In addition, the MWP performances of the integrated PSIC system are characterized, including the link gain, noise figure, receiving sensitivity, and SFDR.
Performance Comparison of Integrated PSIC Schemes
Process | Integration | Phase Reversal | Operating | Cancellation | ||
---|---|---|---|---|---|---|
[ | InP | System | BPD | 400 MHz–6 GHz | 10–100 MHz | |
[ | InP | System | Balun | 670 MHz | 20 MHz | |
[ | SOI | Linear filter | BPD | 5 GHz | 300 MHz | |
[ | SOI | Modulator | DP-MZM | 7–20 GHz | Single frequency | |
This work | SOI | System | 5–25 GHz C, X, Ku, and K bands | 140–630 MHz |
This preliminary research paves the way for the integration of PSIC systems on a silicon photonics platform. Certainly, there is still much work to be done in the future. First, the integrated TODL with a large time delay range and high tuning precision is desired to complete the suppression of interference with high cancellation depth. In this work, cascaded rings operating in the anti-resonant state are adopted to realize continuous time delay tuning with relatively low optical loss. Because only two rings are cascaded, the delay response is not flat enough within the operation band. Additional rings can be applied to overcome the limitation [44]. The switch-based optical delay line is helpful to realize a large delay range without bandwidth restriction for MWP signal processing [45]. The combination of cascaded rings and a switch-based optical delay line can obtain a large range and high tuning precision [46].
Second, the gain of the integrated PSIC system needs to be improved. The insertion loss of the current silicon-based PSIC chip is relatively high, including the absorption loss in the doped silicon waveguide of modulators and coupling loss of the vertical waveguide grating coupler to fiber. Besides optimization of the doping concentration in modulation [47] and the coupling structure for connecting the silicon waveguide and the fiber [48,49], hybrid integration technology [50,51] and the light source and optical amplifier on silicon by using heterogeneous integration or a direct growth method [52,53] provide an efficient way to enhance the gain of the on-chip system.
Third, as far as we know, the currently reported integrated PSIC schemes are mainly about the prototype demonstration of the amplitude, delay, and phase tuning for cancelling a single path interference. In real application scenarios, the interference from transmitting antennas to receiving antennas is a combined result of direct path coupling between the Tx and receiver as well as multiple reflection, scattering, and diffraction through different paths [54]. Therefore, multi-path interference cancellation should be considered. In addition, multi-path interference fluctuates with environmental conditions. Automatic feedback control is essential for implementation of integrated PSIC in full-duplex communications.
Acknowledgment
Acknowledgment. The authors thank Prof. Liu Liu (Zhejiang University) and Prof. Kai-Xuan Chen (South China Normal University) for the advice and assistance on chip design and fabrication.
References
[4] M. Jain, J. I. Choi, T. M. Kim, D. Bharadia, S. Seth, K. Srinivasan, P. Levis, S. Katti, P. Sinha. Practical, real-time, full duplex wireless. Proceedings of the 17th Annual International Conference on Mobile Computing and Networking, 301-312(2011).
[13] M. Huang, D. Zhu, S. Pan. Optical RF interference cancellation based on a dual-parallel polarization modulator. Proceedings of Asia Communications and Photonics Conference, ATh1F.6(2014).
[30] M. David, J. Yao, J. Capmany. Integrated microwave photonics. Nat. Photonics, 13, 80-90(2019).
[34] E. C. Blow, P. Kaul, P. R. Prucnal. Integrated balanced microwave photonic canceller. 2018 IEEE Photonics Conference (IPC), 1-2(2018).
[35] E. C. Blow, C. Huang, Z. Liu, S. J. Markoff, P. R. Prucnal. Silicon photonic weights for microwave photonic canceller. Conference on Lasers and Electro-Optics (CLEO), SW3O.4(2020).
[42] . Universal Mobile Telecommunications System (UMTS); terminal conformance specification; radio transmission and reception (TDD)(2016).
[43] V. J. Urick, J. D. McKinney, K. J. Williams. Fundamentals of Microwave Photonics, 43-52(2015).
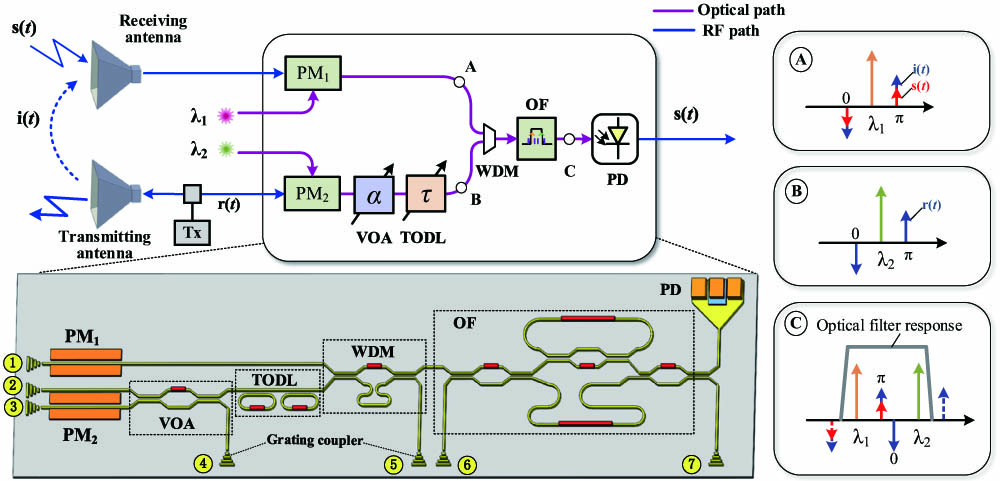
Set citation alerts for the article
Please enter your email address