
- Photonics Research
- Vol. 8, Issue 11, 1776 (2020)
Abstract
1. INTRODUCTION
With the ever-increasing demand for high-speed intra- and inter-data-center connections, optical transceivers with lane rates of 100 Gbit/s are standardized in IEEE Standard 802.3bs [
The works just listed focus on single-channel high-speed transmission. For the silicon platform, monolithic integration, which means complex and/or multi-channel functions are fabricated on the same photonic integrated circuit (PIC) chip, is also an important metric for commercial application [
In this paper, a four-channel SiP MZM chip is integrated with a four-channel driver chip by chip-on-board co-packaging, and a high-speed four-channel SiP transmitter is fabricated. 800 Gbit/s PAM-4 signal transmission and 480 Gbit/s OOK signal transmission using the co-packaged four-channel SiP transmitter are experimentally demonstrated for the first time, to the best of our knowledge. Offline DSP is applied both in the TX side and RX side as pre- and post-compensation to deal with BW limitation and chromatic dispersion (CD) penalty. Different baud rates of PAM-4 signal and OOK signal are modulated on each channel of the transmitter, and the B2B BER is measured to study the uniformity of different channels. Transmission penalty of the PAM-4 and OOK signals for 500 m and 1 km SSMF is then experimentally tested by using one channel of the transmitter, which shows that a 200 Gbit/s PAM-4 signal could achieve 1 km SSMF transmission with the BER lower than the SD-FEC threshold. For the OOK signal, after 500 m and 1 km SSMF transmission, the data rate shrinks to 119 Gbit/s and 118 Gbit/s with SD-FEC threshold. The 800 Gbit/s PAM-4 signal with 1 km transmission is tested, and the BER of each channel is below the SD-FEC threshold.
Sign up for Photonics Research TOC. Get the latest issue of Photonics Research delivered right to you!Sign up now
2. SiP TRANSMITTER DESIGN AND FABRICATION
Figure
Figure 1.(a) Micrograph of the chip-on-board SiP transmitter that co-packaged a four-channel MZM chip and a four-channel driver by wire bonding on the evaluation board, (b) micrograph of the travelling wave electrode, and (c) schematic diagram of the active waveguide’s cross section.
The design and fabrication process of the SiP MZM are improved from our previous work [
Figure 2.EO S21 of the (a) SiP modulator and (b) SiP transmitter co-packaged with the driver.
The four-channel SiGe driver is co-designed with the SiP MZM chip, according to the modulator’s circuit model. To enable more than 100 Gbaud per-lane transmission, the driver is well designed to achieve high BW with our experience in EO co-design [
The driver is wire bonded to the SiP chip on a PCB board. The frequency responses of the co-packaged transmitter are measured and displayed in Fig.
3. SIP TRANSMITTER MEASUREMENT
A. Experiment Setup
The high-speed transmission character of the SiP transmitter is experimentally measured using the setup shown in Fig.
Figure 3.Experiment setup of the PAM-4/OOK signal transmission with TX and RX DSP.
The AWG is connected to the SiP transmitter directly by matched RF cables. In the SiP transmitter, PAM-4/OOK signals are amplified by a co-packaged four-channel driver and then modulated on SiP high-speed MZMs. Two commercial continuous-wave (CW) lasers with output power of and wavelength of are used as the optical source. For each input port, a polarization controller (PC) is inserted between the external laser and SiP transmitter to keep the polarization of input light.
The four-channel modulated signal of the SiP transmitter is coupled out by a four-channel FA with optical power of about for each channel. At the RX side, the optical signal is detected by a commercial high-speed photodetector (PD) after amplification by an erbium-doped fiber amplifier (EDFA). The 6 dB BW of the commercial PD is 60 GHz. Because the commercial PD is packaged without a trans-impedance amplifier (TIA), EDFA is added to enhance the electrical signal with sufficient amplitude. Optical eye diagrams and electrical eye diagrams are measured by a digital signal analyzer (Tektronix DSA 8300 with 70 GHz BW). The detected signal from the PD is digitally captured by a real-time digital storage oscilloscope (DSO) with sampling rate of 256 GS/s (Keysight UXR0704A), and then off-line DSP is carried out by computer for signal demodulation and BER calculation.
In RX-DSP, the captured data are first re-sampled to two samples per symbol (SPS) and filtered by matched RRC filter. Then the data sequence is down-sampled to one SPS and synchronized with original PAM4/OOK bitstreams that are loaded into the AWG. Adaptive time-domain EQ, which is a 51-tap finite-impulse response (FIR) filter based upon the least-mean-square (LMS) error algorithm, is applied for channel estimation and EQ. After the time-domain EQ, the maximum likelihood sequence estimation (MLSE) algorithm in cooperation with an adaptive post-filter is applied to realize faster than Nyquist transmission. An adaptive post-filter could suppress the noise enhanced by time-domain EQ, and the MLSE could eliminate both the residual inter-symbol interference (ISI) of time-domain EQ and the ISI generated by the post-filter. To realize rapid convolution and optimize the factor of filter taps, a training sequence with bit length of 4096 is used both in time-domain EQ and the adaptive post-filter. Finally, BER is calculated for both the PAM-4 signal and OOK signal.
B. B2B BER of Four Channels
The 800 Gbit/s () PAM-4 signal transmission and 480 Gbit/s () OOK signal transmission are first measured in B2B to check the uniformity of different channels. Because we only have one high-speed PD in the lab, the four-channel BERs are tested one by one. The calculated BERs of the PAM-4 signal and OOK signal with respect to different data rates are shown in Figs.
Figure 4.B2B BER with respect to different data rates for the (a) PAM-4 signal and (b) OOK signal.
For the 200 Gbit/s PAM-4 signal, the BER of each channel is around the HD-FEC threshold (e.g., ). As to the 120 Gbit/s OOK signal, the BER of each channel is lower than the SD-FEC threshold (e.g., ). If using the HD-FEC threshold as standard, the data rate could be 118 Gbit/s for the OOK signal. The BER curves for different channels both in Figs.
Figure 5.B2B eye diagrams plotted in DSP with different baud rates after time-domain EQ for the (a) PAM-4 signal and (b) OOK signal.
C. 500 m and 1 km Fiber Transmission
The BERs after the 500 m and 1 km SSMF transmission are also measured for both the PAM-4 signal and OOK signal to check the transmission penalty. Because the uniformity of the four channels was proven to be good in Section 2, the BER penalty of 500 m and 1 km transmission is illustrated using just one channel (channel 3). The BERs of the PAM-4 signal with different data rates after 500 m and 1 km SSMF transmission are shown in Fig.
Figure 6.BERs with respect to different data rates for the (a) PAM-4 signal and (b) OOK signal after 500 m and 1 km SSMF transmission.
The 800 Gbit/s () PAM-4 signal transmission over 1 km SSMF was measured, and the BERs of four channels are plotted in Fig.
Figure 7.BERs of all four-channel PAM-4 signals with respect to different data rates after 1 km SSMF transmission.
4. CONCLUSION
We experimentally demonstrate the transmission of an 800 Gbit/s PAM-4 signal up to 1 km SSMF based on a four-channel integrated SiP transmitter. The SiP transmitter is fabricated by co-packaging a four-channel high-speed silicon TW-MZM chip with a four-channel driver chip. The 3 dB BWs of the silicon MZM chip and the co-packaged transmitter are over 60 GHz and 40 GHz, respectively. DSP is implemented both at the TX side and RX side, which is necessary to compensate for the BW limitation and CD with 500 m/1 km SSMF transmission. The B2B BERs for 800 Gbit/s () PAM-4 signal transmission and 480 Gbit/s () OOK signal transmission are experimentally tested, and they show good uniformity of performance between different channels. Experimental results show that the 800 Gbit/s PAM-4 signal could be achieved for B2B and 1 km SSMF transmission with the HD-FEC threshold and SD-FEC threshold, respectively. The 480 Gbit/s OOK signal with B2B BER is below the SD-FEC threshold. However, after 500 m and 1 km SSMF transmission, the data rate of the single-channel OOK slightly shrinks to 119 Gbit/s and 118 Gbit/s, which means the CD penalty is tolerable.
References
[2] M. Bohn, P. Magill, M. Hochberg, D. Scordo, A. Novack, M. Streshinsky. Next-generation silicon photonic interconnect solutions. Optical Fiber Communication Conference (OFC), M3J.3(2019).
[4] H. Mardoyan, F. Jorge, O. Ozolins, J. M. Estaran, A. Udalcovs, A. Konczykowska, M. Riet, B. Duval, V. Nodjiadjim, J. Dupuy, X. Pang, U. Westergren, J. Chen, S. Popov, S. Bigo. 204-GBaud on-off keying transmitter for inter-data center communications. Optical Fiber Communication Conference (OFC), Th4A.4(2018).
[5] S. M. R. Motaghiannezam. Optical PAM4 signaling and system performance for DCI applications. Optical Fiber Communication Conference (OFC), M3A.1(2019).
[7] T. Wettlin, T. Rahman, J. Wei, S. Calabrò, N. Stojanovic, S. Pachnicke. Comparison of PAM formats for 200 Gb/s short reach transmission systems. Optical Fiber Communications Conference and Exhibition (OFC), Th2A.37(2020).
[8] Y. Zhang, M. Xu, H. Zhang, M. Li, J. Jian, M. He, L. Chen, L. Wang, X. Cai, X. Xiao, S. Yu. 220 Gbit/s optical PAM4 modulation based on lithium niobate on insulator modulator. European Conference on Optical Communication (ECOC), PD2.6(2019).
[10] J. Zhang, J. Yu, L. Zhao, K. Wang, J. Shi, X. Li, M. Kong, W. Zhou, X. Pan, B. Liu, X. Xin, L. Zhang, Y. Zhang. Demonstration of 260-Gb/s single-lane EML-based PS-PAM-8 IM/DD for datacenter interconnects. Optical Fiber Communication Conference (OFC), W4I.4(2019).
[15] M. Jacques, Z. Xing, A. Samani, X. Li, E. El-Fiky, S. Alam, O. Carpentier, P. Koh, D. V. Plant. Net 212.5 Gbit/s transmission in O-band with a SiP MZM, one driver and linear equalization. Optical Fiber Communication Conference Postdeadline, Th4A.3(2020).
[17] Y. Ma, C. Williams, M. Ahmed, A. Elmoznine, D. Lim, Y. Liu, R. Shi, T. Huynh, J. Roman, A. Ahmed, L. Vera, Y. Chen, A. Horth, H. Guan, K. Padmaraju, M. Streshinsky, A. Novack, R. Sukkar, R. Younce, A. Rylyakov, D. Scordo, M. Hochberg. An all-silicon transmitter with co-designed modulator and DC-coupled driver. Optical Fiber Communication Conference (OFC), Tu2A.2(2019).
[19] H. Yu, P. Doussiere, D. Patel, W. Lin, K. Al-Hemyari, J. Park, C. Jan, R. Herrick, I. Hoshino, L. Busselle, M. Bresnehan, A. Bowles, G. A. Ghiurcan, H. Frish, S. Yerkes, R. Venables, P. Seddighian, X. Serey, K. Nguyen, A. Banerjee, S. A. Asl, Q. Zhu, S. Gupta, A. Fuerst, A. Dahal, J. Chen, Y. Malinge, H. Mahalingam, M. Kwon, S. Gupta, A. Agrawal, R. Narayan, M. Favaro, D. Zhu, Y. Akulova. 400 Gbps fully integrated DR4 silicon photonics transmitter for data center applications. Optical Fiber Communication Conference (OFC), T3H.6(2020).
[24] N. Qi, X. Xiao, S. Hu, M. Li, H. Li, Z. Li, P. Y. Chiang. A 32 Gb/s NRZ, 25 GBaud/s PAM4 reconfigurable, Si-photonic MZM transmitter in CMOS. Optical Fiber Communications Conference and Exhibition (OFC), Th1F.3(2016).
[26] H. Ahmed, D. Lim, A. Elmoznine, Y. Ma, T. Huynh, C. Williams, L. Vera, Y. Liu, R. Shi, M. Streshinsky, A. Novack, R. Ding, R. Younce, R. Sukkar, J. Roman, M. Hochberg, S. Shekha, A. Rylyakov. 30.6 A 6 V swing 3.6% THD >40 GHz driver with 4.5× bandwidth extension for a 272 Gb/s dual-polarization 16-QAM silicon photonic transmitter. IEEE International Solid-State Circuits Conference (ISSCC), 484-486(2019).
[28] E. Temporiti, G. Minoia, M. Repossi, D. Baldi, A. Ghilioni, F. Svelto. 23.4 A 56 Gb/s 300 mW silicon-photonics transmitter in 3D-integrated PIC25G and 55 nm BiCMOS technologies. IEEE International Solid-State Circuits Conference (ISSCC), 404-405(2016).
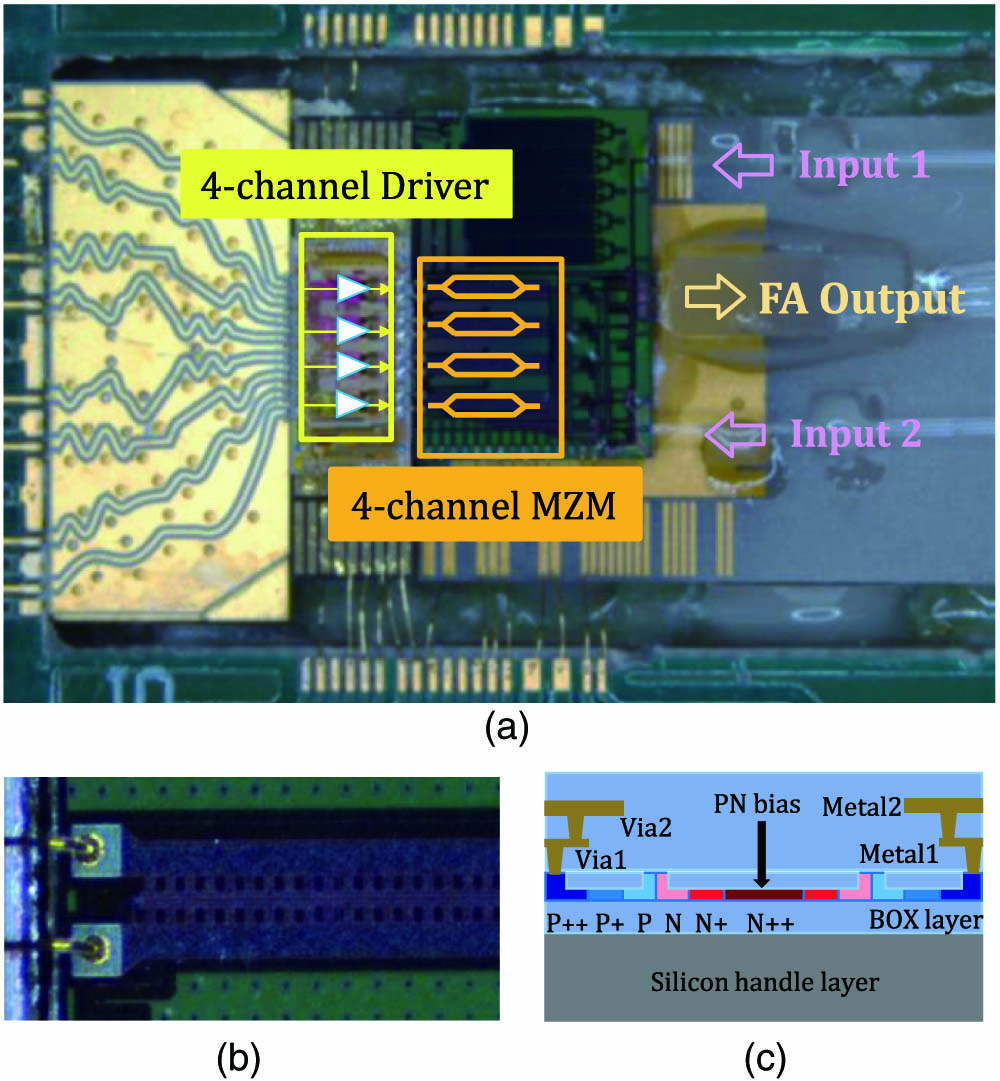
Set citation alerts for the article
Please enter your email address