Abstract
We explored Q-switching mechanism for the newly proposed Tm/Ho composite laser via developing a hybrid resonator for separating the intra-cavity Tm laser modulated by the saturable absorber (SA). With a Cr:ZnSe SA, successful passively Q-switching process with the maximum average output power of 474 mW and the shortest pulse width of 145 ns were obtained at the pulse repetition frequency of 7.14 kHz, where dual wavelength oscillation in both 2090 nm and 2097 nm was observed. This work provides an effective way for a direct laser diode (LD) pumped Q-switched Ho laser, which is compact and accessible. Furthermore, the current SA could be replaced by the 2D materials with broadband saturable absorption such as topological insulators or transition-metal dichalcogenides for seeking novel PQS lasers.Introduction
Within the transparent window of atmosphere and the strong absorption band of water, Ho lasers have a series of important applications in fields such as surgeries, mid-infrared super-continuum, and lidar systems1-3, which have received more and more attentions. Particularly, pulsed Ho lasers with the wavelength around 2.1 μm are efficient pump sources for mid-infrared frequency conversion toward the 3~12 μm molecular fingerprint region4 via optical parameter oscillation (OPO) or differential frequency generation (DFG)5, 6, owning to the wavelength farther away from absorption edge of the famous mid-infrared crystals ZPG and OP:GaAs than other Tm or Ho laser wavelengths7.
Due to the lack of absorption band of Ho3+ at 800 nm, Ho lasers are commonly realized via the Tm, Ho co-doped mechanism8 or intra-cavity pumping manner9 for directly utilizing the mature AlGaAs LDs. However, the above lasers have limitations: attributing to the cooperative up-conversion process between the Tm3+ and Ho3+ ions, the Tm, Ho co-doped lasers should be cooled by cryogenic devices for Watt-level operation. Rigorous calibration between the separately cooled Tm-doped and Ho-doped gain media should be maintained for the intra-cavity pumped Ho lasers, which complicated the system setup. Hence, with the development of 1.9 μm lasers, such as Tm lasers or 1.9 μm LD, in-band pumping became a major way to realize Ho lasers for the past two decades10-12. However, the 1.9 μm pump sources are more expensive than common AlGaAs LDs and the setup is bulky especially when using the Tm lasers due to the cascade pumping scheme13.
With the development of 2 μm saturable absorbers, such as 1D materials (single wall carbon nanotube and gold nanorod)14, 15, 2D materials (graphene, topological insulators, and ternary chalcogenides)16-18, and transition metal ion-doped Ⅱ-Ⅳ crystals (Cr2+:ZnSe, Cr2+:ZnS)19, 20, passively Q-switching (PQS) in 2 μm Tm-doped and Ho-doped lasers has become a hot topic, which is characterized by compactness, easy implementation, and cost-saving compared with the actively Q-switching (AQS) manners. Among the above SAs, Cr2+:ZnSe/ZnS crystals were demonstrated to be robust for achieving higher pulse energy and better PQS stability19, owing to the mature crystal growth process with fewer defects. Although both PQS and AQS were well demonstrated in in-band pumped and Tm, Ho co-doped Ho lasers12, 21, Q-switching in intra-cavity pumped Ho lasers were limited due to the saturable effect in Ho-doped gain medium22, 23, which led to a disorder pulse burst and the failure in Q-switching.
Recently, via integrating the Tm-doped and Ho-doped gain medium into a single bulk structure, the Tm/Ho composite laser has been realized and demonstrated to meet the requirement of 2.1 μm lasers in compactness, accessibility, and robustness24, which could be pumped by LDs with wavelength from 760 nm to 820 nm at broad room-temperatures25. However, pulse properties of such a Ho laser mechanism haven't been explored yet. On the other hand, saturable effect in both Tm-doped and Ho-doped regions of the composite gain medium will seriously challenge a successful Q-switching process due to the same resonant-pumping manner as the intra-cavity pumped Ho lasers. Hence, it is of significance to explore and realize Q-switching for the newly proposed Tm/Ho composite laser.
In this paper, crystalline Cr2+:ZnSe crystal was selected as SA for exploring PQS properties of the Tm/Ho composite laser. Successful PQS process with the maximum output power of 474 mW and shortest pulse width of 145 ns was realized in a hybrid cavity structure, which could separate the intracavity Tm laser form Ho laser efficiently before modulated by the SA and in avoiding the cease in laser oscillation. The ideas here could also be adopted to realize PQS in the Tm/Ho composite lasers modulated by other SAs prepared with the 1D or 2D materials for seeking novel pulse Ho lasers.
Experimental setup
The schematic of the experiment is depicted in Fig. 1. A Tm/Ho:YAG crystal integrated via diffusion bonding the 3.5 at.% Tm:YAG and 0.6 at.% Ho:YAG crystals into a single bulk structure was served as the gain medium. The composite gain medium had a dimension of 3 mm×3 mm×14 mm with an 8 mm long Tm-doped region, which was wrapped by indium foil and mounted into a copper heat sink for water cooling at 16 ℃. A concave-plano cavity with a physical length of 60 mm was applied to make sure the smallest cavity mode size on the output coupler M2. M1 was a plano-concave mirror with radius of curvature of 100 mm, which was coated anti-refection (AR, T > 97%) at 750-850 nm and high-reflection (HR, R > 99.5%) at 1.9-2.2 μm. M2 was a plano-plano output coupler, which was HR at emission band of the Tm laser (R > 99% at 1.9-2.02 μm) and had transmittance of 10% at Ho laser (T≈10% at 2.09-2.2 μm) respectively.
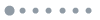
Figure 1.Layout of the PQS Tm/Ho composite laser.
The Cr2+:ZnSe SA with the dimension of 5 mm×5 mm×1.7 mm and initial transmittance of 92% at 2.1 μm was mounted into a copper heat sink and placed close to M2 for tight focusing operation26. For separating the intra-cavity Tm laser from modulated by the SA, a fused silica spectral filter F1 AR coated at 2.09-2.12 μm (T > 95%) and HR coated at 1.9-2.02 μm (R > 93%) were inserted inside the cavity to form a hybrid cavity structure. A fiber coupled 808 nm LD (NL-PPS50, Nligt Inc.) with a core diameter of 400 μm and numerical aperture of 0.22 was applied as the pump source. L1 and L2 are identical plano-convex mirrors with a focus length of 40 mm for forming a pump waist radius of 213 μm inside the composite gain medium, which was calculated by the ABCD matrix. M3 is a dichromatic mirror applied for filtering the Ho laser from the 800 nm pump light. The average output power was measured by an Ophir power meter (30(150)A-LP1-18, Ophire Inc.), and the lasing wavelength by a mid-IR spectrum analyzer (771A-IR, Bristol Instruments Inc.). Pulse properties of PQS composite Ho laser were analyzed by a 2 GHz bandwidth oscilloscope (MSO 2034, Tektronix Inc.) connected with an InGaAs detector (DET05D/M, Thorlabs Inc).
Resonator for PQS Tm/Ho composite laser
According to the model for PQS lasers27, key threshold criterion for the PQS process is
where σgs is ground state absorption cross section of the SA, σ is gain cross section of the Ho-doped region, ωs and ωc are cavity mode radii of the Ho laser on the composite gain medium and the SA, respectively. ni and nth are dimensionless parameters meeting
where T0 is initial transmittance of the SA, TOC is transmittance of the output coupler, L is the round-trip cavity loss, and β is ratio between σgs and the excited state absorption cross section σes of the SA.
In the concave-plano cavity for the Tm/Ho composite laser described by g parameters25
Cavity mode waist ωs and ωc can be written as
where ${l_{\rm{e}}} = {l_{\rm{1}}} + {l_{\rm{2}}} + \frac{{{l_{\rm{r}}}}}{n} - D({l_{\rm{1}}} + \frac{{{l_{\rm{r}}}}}{{2n}})({l_{\rm{2}}} + \frac{{{l_{\rm{r}}}}}{{2n}})$
is the equivalent cavity length, l0= 60 mm is length of the concave-plano cavity, Ri is curvature of radius of mirror Mi, li is distance between the gain medium and Mi. lr, n, and D are the length, refractive index, and thermal lens of the composite gain medium.
As described in Ref.28, D is proportional to the absorbed pump power Pabs and inverse proportional to the pump area as D(Pabs)=CPabs/ωp2, where C is 8.2893 mm/W according to the calculation result in our previous work25. Hence, with other given cavity parameters, ωs and ωc are variables of Pabs, as shown in Figs. 2(a) and 2(b). As denoted in Fig. 3, peak gain cross section σ of the Ho-doped region is 1.4×10-20 cm2. For the Cr2+:ZnSe SA, σgs and σes are 1.3×10-19 cm2 and 0.9×10-19 cm2 respectively29. Inserting values of σ, σgs, and σes into Equation (2) and initializing L as 0.03, with the calculated ωs and ωc, evolution in the key parameter α in Equation (1) with Pabs is depicted in Fig. 2(c), where ${\alpha _{{\rm{th}}}} = \frac{{{n_{\rm{i}}}}}{{{n_{{\rm{th}}}}}}{\left( {\frac{{{n_{\rm{i}}}}}{{{n_{{\rm{th}}}}}} - 1} \right)^{ - 1}}$ denotes the threshold value. As shown in Fig. 2(c), the higher curvature radius R1 leads to the lager stable region and the smaller α, which is not suit for more stable PQS process according to threshold criterion. Although R1=75 mm contributes for the largest cavity mode size in the gain medium and the smallest mode size in SA for tight focusing among R1 of 75 mm, 100 mm and 200 mm, the shortest stable region denoted in Fig. 2(c) limits the achievable maximum output power for the PQS Ho laser. Therefore, M1 with R1 of 100 mm was applied in the following experiment, which brings moderate α and higher unstable threshold at approximately 6.5 W.
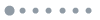
Figure 2.(a, b) Evolutions in cavity mode sizes ωc and ωs with the absorbed LD power Pabs at different M1 curvature radii (R1=75 mm, 100 mm and 200 mm); (c) Evolution in key parameter α with Pabs, where the colorful areas denote corresponding unstable regions (green for R1=75 mm, blue for R1=100 mm), αth is (ni/nth)/(ni/nth-1) in Equation (1). Values of the parameters for the above calculation are summarized in Table 1.
Parameter | Value |
σgs | 1.3×10-19 cm2, Ref.29 |
σ | 1.4×10-20 cm2 |
σes | 0.9×10-19 cm2, Ref.29 |
C | 8.2893 mm/W, Ref.25 |
T0 | 92% |
λ | 2122 nm |
L | 0.03 |
ωp | 213 μm |
TOC | 10% |
R2 | Infinity |
R1 | 75 mm, 100 mm, 200 mm |
l1 | 8 mm |
l2 | 38 mm |
n | 1.82 |
Table 1. Values of the parameters in Equations (1)–(5).
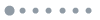
Figure 3.Emission cross section of the Tm-doped and Ho-doped regions and transmittance of the Cr:ZnSe SA.
Results and discussion
The start of PQS
As shown in Fig. 3, emission bands of the intra-cavity Ho laser and Tm laser are within the designed initial transmittance curve of the SA. Due to the smaller gain cross section of Tm3+ ion compared with that of Ho3+ ion, oscillation in Tm laser was limited to sufficiently pump the Ho-doped region under both absorption losses from the Ho-doped region and the SA. Therefore, no oscillation in the PQS Tm/Ho composite laser was observed when directly inserted and adjusted the SA inside the laser cavity as normal PQS lasers.
As shown in Fig. 1, the spectral filter F1 was inserted into the cavity for preventing the Tm laser from being modulated and ceased by the SA and making sure the Ho laser oscillation. In the hybrid cavity consisted with M1, M2 and F1, characteristic pulse train of the free running Tm/Ho composite laser without SA is depicted in Fig. 4(a), which was irregular and unstable with time during the power scaling process. Stable PQS process occurred when inserting the SA into the hybrid cavity, where typical pulse train with the pulse repetition frequency (PRF) of 850 Hz and pulse width of 218 ns was shown in Fig. 4(b). Under the same absorbed LD power of 3.49 W, pulse train in Fig. 4(b) was regular with higher signal intensity compared with that in Fig. 4(a).
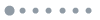
Figure 4.Comparison in pulse trains from the hybrid cavity before (a) and after (b) using the Cr2+:ZnSe.
The laser properties
Before inserting the Cr:ZnSe SA inside the hybrid cavity, free running properties were measured first with the maximum output power of 667 mW and slope efficiency of 25.9% considering the absorbed LD power. As shown in Fig. 5(a), using the SA, the maximum average power decreased to be 474 mW at an absorbed LD power of 5.67 W with a slope efficiency of 20.3% and the lasing threshold increased from 3.04 W to 3.28 W due to the insertion loss from the SA. Beam quality of the PQS Ho laser at the maximum output power was measured to be with Mx2=1.22 and My2=1.15 in the horizontal and vertical directions, respectively, via using a beam quality analyzer (Nanomode scan, Ophire Inc.), where the corresponding beam profile with a concentric 3D beam shape at the beam waist was depicted in Fig. 5(b).
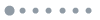
Figure 5.(a) Average output powers of the free-running Ho laser and PQS Ho laser versus absorbed pump power. (b) Beam profile of the PQS composite Ho laser at the maximum output power of 474 mW (Inset: 3D beam profile at the beam waist).
Lasing wavelength for the free-running Ho laser was stabilized at approximately 2122.2 nm during the power scaling process, which is the same as that from a simple plano-concave cavity in our previous work25, where the characteristic spectrum at the maximum output power of 667 mW was shown in Fig. 6(a). During the PQS process, the wavelength at 2090 nm oscillated first (Fig. 6(b)) before scaling up the output power above 200 mW, where dual wavelength oscillation occurred at both 2090 nm and 2097 nm as shown in Fig. 6(c). Figure 6(d) illustrates the evolutions in Ho laser wavelengths at different output powers operating at free-running and PQS processes respectively. The measured Ho laser wavelengths were corresponding to the emission peaks of the Ho-doped region in Fig. 3, which switched from 2122 nm to shorter wavelengths due to the introduced cavity loss from the SA30.
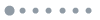
Figure 6.Wavelength properties of the Tm/Ho composite laser:
(a) at the maximum free-running (CW) laser power; (b) at the threshold PQS laser power of 20 mW; (c) at the maximum PQS laser power; (d) the measured peak wavelengths at different average output power.
Evolution in PRF and pulse width for the PQS composite Ho laser were measured and depicted in Fig. 7. Increasing the average output power from 20 mW to 474 mW, PRF increased from 120 Hz to 7.14 kHz with the pulse width decreased from 229 ns to 145 ns correspondingly. Typical pulse train at the maximum PRF of 7.14 kHz was depicted in Fig. 8(a). Figure 8(b) shows the detail view of typical pulse profile from Fig. 8(a), which has a pulse width of 145 ns and corresponds to a pulse energy and a peak power of 66 μJ and 458 W, respectively.
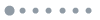
Figure 7.Evolutions in the pule repetition frequency and pulse width with the absorbed LD power.
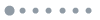
Figure 8.(a) Typical pules train with PRF of 7.14 kHz at the maximum PQS output power. (b) Detail view of the shortest pulse with a width of 145 ns at the maximum output power.
Conclusions
In conclusion, we have demonstrated Q-switching properties of the recently proposed Tm/Ho composite laser, which met failure when following the traditional PQS manner due to the introduced modulation losses in both the intra-cavity Tm laser and Ho laser. A concave-plano hybrid resonator was designed for realizing a successful PQS process through filtering the intra-cavity Tm laser from Ho laser efficiently, where the maximum output power of 474 mW with a pulse width of 145 ns and a pulse energy of 66 μJ at PRF of 7.14 kHz was obtained. Through paving a way for achieving Q-switching in the Tm/Ho composite mechanism, this work tries to provide a compact and accessible pulse Ho laser which facilitates the direct use of common AlGaAs LDs. Moreover, the current SA can be replaced by the popular 2D materials, such as topological insulator, ternary chalcogenides, or graphene for exploring novel PQS Tm/Ho composite Ho lasers.
Acknowledgements
We are grateful for financial supports from National Key Research and Development Program of China (Grant No. 2017YFB1104500), Natural National Science Foundation of China (NSFC) (Grant No. 61875200), and China Postdoctoral Science Foundation (Grant No. 2018M642575)
Competing interests
The authors declare no competing financial interests.
References
[1] S Hein, R Petzold, M Schoenthaler, U Wetterauer, A Miernik. Thermal effects of Ho: YAG laser lithotripsy: real-time evaluation in an in vitro model. World J Urol, 36, 1469-1475(2018).
[2] J W Zhang, Mak K Fai, N Nagl, M Seidel, D Bauer et al. Multi-mW, few-cycle mid-infrared continuum spanning from 500 to 2250 cm-1. Light Sci Appl, 7, 17180(2018).
[3] K Mizutani, S Ishii, M Aoki, H Iwai, R Otsuka et al. 2 μm Doppler wind lidar with a Tm:fiber-laser-pumped Ho:YLF laser. Opt Lett, 43, 202-205(2018).
[4] A Schliesser, N Picque, T W Hansch. Mid-infrared frequency combs. Nat Photonics, 6, 440-449(2012).
[5] T Kanai, P Malevich, S S Kangaparambil, K Ishida, M Mizui et al. Parametric amplification of 100 fs mid-infrared pulses in ZnGeP2 driven by a Ho:YAG chirped-pulse amplifier. Opt Lett, 42, 683-686(2017).
[6] A Hemming, J Richards, A Davidson, N Carmody, S Bennetts et al. 99 W mid-IR operation of a ZGP OPO at 25% duty cycle. Opt(2013).
[7] Nonlinear Optics 2017 (Optical Society of America, 2017); http://doi.org/10.1364/NLO.2017.NTu2A.1.
[8] 2 OPO. In Advanced Solid State Lasers 1998 (Optical Society of America, 1998); http://doi.org/10.1364/ASSL.1998.FC1.
[9] C Bollig, R A Hayward, W A Clarkson, D C Hanna. 2-W Ho:YAG laser intracavity pumped by a diode-pumped Tm:YAG laser. Opt Lett, 23, 1757-1759(1998).
[10] Advanced Solid State Lasers 1998 (Optical Society of America, 1998); http://doi.org/10.1364/ASSL.1998.ML4.
[11] H Chen, D Y Shen, J Zhang, H Yang, D Y Tang et al. In-band pumped highly efficient Ho:YAG ceramic laser with 21 W output power at 2097 nm. Opt Lett, 36, 1575-1577(2011).
[12] Y X Zhang, C Q Gao, Q Wang, Q X Na, M Zhang et al. Single-frequency, injection-seeded Q-switched Ho:YAG ceramic laser pumped by a 1.91 μm fiber-coupled LD. Opt Express, 24, 27805-27811(2016).
[13] S Lamrini, P Koopmann, M Schäfer, K Scholle, P Fuhrberg. Directly diode-pumped high-energy Ho:YAG oscillator. Opt Lett, 37, 515-517(2012).
[14] M Chernysheva, C B Mou, R Arif, M AlAraimi, M Rümmeli et al. High power Q-switched thulium doped fibre laser using carbon nanotube polymer composite saturable absorber. Sci Rep, 6, 24220(2016).
[15] H T Huang, M Li, P Liu, L Jin, H Wang et al. Gold nanorods as the saturable absorber for a diode-pumped nanosecond Q-switched 2 μm solid-state laser. Opt Lett, 41, 2700-2703(2016).
[16] T Zhao, Y Wang, H Chen, D Y Shen. Graphene passively Q-switched Ho:YAG ceramic laser. Appl Phys B, 116, 947-950(2014).
[17] X Liu, K Yang, S Zhao, T Li, W Qiao et al. High-power passively Q-switched 2 μm all-solid-state laser based on a Bi2Te3 saturable absorber. Photonics Res, 5, 461-466(2017).
[18] B Z Yan, B T Zhang, J L He, H K Nie, G R Li et al. Ternary chalcogenide Ta2NiS5 as a saturable absorber for a 1.9 μm passively Q-switched bulk laser. Opt Lett, 44, 451-454(2019).
[19] B Cole, L Goldberg. Highly efficient passively Q-switched Tm:YAP laser using a Cr:ZnS saturable absorber. Opt Lett, 42, 2259-2262(2017).
[20] J L Lan, B Xu, Y Z Zhang, H Y Xu, Z P Cai et al. Tunable and passively Q-switched laser operation of Tm:CaYAlO4 between 1848 nm and 1876 nm. Opt Laser Technol, 109, 33-38(2019).
[21] L J Li, X N Yang, L Zhou, W Q Xie, Y L Wang et al. Active/passive Q-switching operation of 2 μm Tm, Ho:YAP laser with an acousto-optical Q-switch/MoS2 saturable absorber mirror. Photonics Res, 6, 614-619(2018).
[22] M Schellhorn, A Hirth, C Kieleck. Ho:YAG laser intracavity pumped by a diode-pumped Tm:YLF laser. Opt Lett, 28, 1933-1935(2003).
[23] X F Yang, H T Huang, D Y Shen, H Y Zhu, D Y Tang. 2.1 μm Ho:LuAG ceramic laser intracavity pumped by a diode-pumped Tm:YAG laser. Chin Opt Lett, 12, 121405(2014).
[24] H Z Huang, J H Huang, Y Ge, H Zheng, W Weng et al. 2.1 μm composite Tm/Ho:YAG laser. Opt Lett, 43, 1271-1274(2018).
[25] H Z Huang, J Deng, Y Ge, J H Li, J H Huang et al. Direct 800 nm diode-pumped Holmium laser with broad pump wavelength range and temperature adaptability. Opt Express, 27, 13492-13502(2019).
[26] Y J Huang, Y P Huang, P Y Chiang, H C Liang, K W Su et al. High-power passively Q-switched Nd:YVO4 UV laser at 355 nm. Appl Phys B, 106, 893-898(2012).
[27] P H Tuan, C C Chang, F L Chang, C Y Lee, C L Sung et al. Modelling end-pumped passively Q-switched Nd-doped crystal lasers: manifestation by a Nd:YVO4/Cr4+:YAG system with a concave-convex resonator. Opt Express, 25, 1710-1722(2017).
[28] Y T Chang, Y P Huang, K W Su, Y F Chen. Comparison of thermal lensing effects between single-end and double-end diffusion-bonded Nd:YVO4 crystals for 4F3/2→4I11/2 and 4F3/2→4I13/2 transitions. Opt Express, 16, 21155-21160(2008).
[29] 2+: ZnSe. In Advanced Solid State Lasers 2000 (Optical Society of America, 2000); http://doi.org/10.1364/ASSL.2000.MC7.
[30] N P Barnes, F Amzajerdian, D J Reichle, W A Carrion, G E Busch et al. Diode pumped Ho:YAG and Ho:LuAG lasers, Q-switching and second harmonic generation. Appl Phys B, 103, 57-66(2011).